Hyperglycemia
Obesity
Inflammation
Liver
↑ Glucose production
↑ Insulin
↑ ROS
↑ AGE
↓ Fatty acid oxidation
↑Hyperglycemia
↑Insulin resistance
↑Cytokines/chemokines
↑Fatty acids (fatty liver)
↑ Innate immune cells
↓ Glucose production
↓ Insulin degradation/sens.
↑ Immune cell adhesion
↑ Apoptosis (↑ damage)
↑ Fibrosis (↓ response)
↑ IgG & complement
Brain
↑ Glucose consumption
↑Appetite suppression
↑ROS
↓Appetite suppression
↓Glucose consumption
↓ Insulin degradation/sens.
↑ IgG deposits
↑ Amyloid deposits
Pancreas
↑Insulin production
↑Insulin production
↓Insulin production
↓Glucose sens.
↑ Immune cell adhesion
↑ Apoptosis (↑ damage)
↑ Fibrosis (↓ response)
↑IgG & complement
↑ Auto-immune response
Adipose
↑ Adipokine response
↑ Energy storage
↓ Lipolysis
↑ FFA/TG
↓Adipokine response
↑Cytokines/chemokines
↑Hypoxia
↑Lipid loading
↓Lipolysis
↓ Insulin sens.
↑ Immune cell adhesion
↑ Apoptosis (↑ damage)
↑ Fibrosis (↓ response)
↑ IgG & complement
Muscle
↑ Glucose uptake
↑ Mitochondria
↓Fatty acid oxidation
↑ROS
↑AGE
↑Kallikrein (Kinins)
↑RAGE
↓ Glucose uptake
↑ IgG-CTF generation
↓Glucose consumption
↓Insulin degradation/sens.
↑ Immune cell adhesion
↑ Apoptosis (↑ damage)
↑ Fibrosis (↓ response)
Vascular system
↑ Vascular dysfunction
↓ NO (↑ROS)
↓ NO (↓eNOS by insulin)
↑Kinins
↑AGE (HbA1c)
↑glyCD59 (complement)
↑RAGE
↑ Vascular dysfunction
↓ NO (↑cytokines)
↑ Apidose AgnII
↑ Immune cell adhesion
↑ eNOS (AMBK)
↑ Vascular dysfunction
↑ Immune cell adhesion
↑ Fibrosis (↓elasticity)
↑ Membrane thickening
↑ Apoptosis
↑ Coagulation
↑ Complement
11.2 Hyperglycemia Stress Markers
It has been clearly shown that hyperglycemia correlates to diabetic inflammation, complications, and worsened progression to end stage disease. Under normal conditions, hyperglycemia induces insulin secretion by the pancreas (Table 11.1). Insulin reduces production of glucose and fatty acid oxidation by the liver as well as glucose stored by muscle (Akt/PIK3 pathway), and it decreases adipose energy storage and lipolysis. Glucose consumption by the brain and red blood cells is stimulated by glucose transport (GLUT1/4), and appetite suppression is signaled by the brain.
Insulin resistance is an early indicator of diabetic conditions, and this impairment leads to hyperglycemia. As such, glucose measurement remains the primary tool relied upon for diabetes diagnosis and monitoring. During fasting, the liver is the sole source of glucose production with the major route being gluconeogenesis (production of new glucose from noncarbohydrate precursors) and the minor route being glycogenolysis (break down of glycogen) Adipose is the major energy store of noncarbohydrate precursors (e.g. triglycerides lipolysis to free fatty acids and glycerol) for gluconeogenesis, and the brain and red blood cells are the major consumers of glucose. These organs normally act in concert, and impaired insulin metabolism leads to hyperglycemia stress across all organs along with reduced fatty acid metabolism. Hyperglycemia stress increases reactive oxidative species (ROS), advanced glycation end products (AGE), vascular dysfunction, and complement activation in the key tissues.
Measurements of glucose, insulin, and glycated hemoglobin (HbA1c) are essential tools to manage hyperglycemia (Table 11.2) where the goal of hyperglycemic management is to use diet, exercise, and medications to obtain glucose values in a normal range. Insulin resistance is often the underlying cause of an inability to manage hyperglycemia. As reviewed in Chap. 1, many factors impact and correlate with insulin sensitivity. Insulin degradation is providing new understandings of insulin sensitivity. The direct or indirect measurement of insulin resistance is difficult to obtain and is best estimated using the traditional methods. However, the oral glucose tolerance test (OGTT) is not a spot test, and HbA1c is a late marker for prediction of diabetes in a group of prediabetes patients, and diabetes cannot be predicted from a single new marker. This reduces the ability to identify patients who are likely to progress to diabetes and respond to therapy. Additionally, hormones such as growth hormone growth hormone (GH), insulin-like growth factors (IGF), IGF binding proteins (IGFBP), epinephrine, glucagon, and cortisone impact the glucose metabolic pathways.
Table 11.2
Markers of glycation stress
Measurement | Markers | Key cellular pathways |
---|---|---|
Insulin resistance/sensitivity | Glucose | AKT-PIK3 |
Insulin | GLUT1/4 | |
C peptide | IDE | |
Glycemic control | HbA1c | Membrane glycation |
Glycemic control & complement stress | Glycated CD59 | MAC (complement activation) |
Advanced glycation end products (AGE) such as HbA1c have shown value for measurement of glycemic control over periods of 3–4 months. Whereas, other nontraditional AGE assays, such as glycated albumin, fructosamine, and 1,5-anhydroglucitol (1,5-AG), which measure glycemic control over a shorter period, have not proved more valuable than HbA1c (Beck et al. 2011). Correlations of complications with nontraditional AGE assays are typically less significant.
All current AGE assays lack a direct mechanistic link to complications, and the receptors for advanced glycation end products (RAGE) have not provided that mechanistic link. While insulin increases the receptors for AGE (RAGE), activation of RAGE requires immune cell activation, and sRAGE levels do not correlate with glycemic control or complications (Lam et al. 2013; Motawi et al. 2013). The formation of AGE on glomerular basement membrane (GBM) directly correlates with HbA1c but is not linked to tissue damage or cellular proliferation without immune cell involvement. Next generation AGE markers such as glycated CD59 offer hope of a true mechanistic link (Chap. 2). Glycated CD59 could offer improvement over HbA1c by its correlation with glucose intolerance in short term glycemic control and by offering a molecular connection that is tied to a direct cell death pathway, as exemplified in the literature by an increased interest in the formation of membrane attack complex (MAC) in the compliment cascade (Qin et al. 2004).
Many therapeutic approaches have been allied to lowering hyperglycemia (Mazzola 2012), and insulin is by far the most effective means used. Other approaches include sulfonylureas and glinides, which lower glucose by enhancing insulin secretion; metformin which suppresses glucose production in the liver; α-glucosidase inhibitors, which reduce the rate of digestion of polysaccharides in the small intestine; thiazolidinediones (TZD), which activate PPARs (peroxisome proliferator-activated receptors) to increase insulin sensitivity of muscle, fat, and liver; incretins of the secretin family of hormones like Glucose-dependent insulinotropic polypeptide (GIP) and glucagon-like peptide-1 (GLP-1), which improve hyperglycemia by indirectly stimulating insulin secretion and suppressing glucagon secretion; dipeptidyl peptidase-4 (DPP-4) inhibitors, which prolong incretin half-life; amylin agonists, which act as a neuroendocrine hormone to enhance satiety and reduce food intake; and sodium-glucose transporter 2 (SGLT2) inhibitors, which reduce glucose reabsorption in the proximal tubule of the kidney.
11.3 Markers of Oxidative Stress
While it appears clear that hyperglycemia leads to oxidative stress damage in tissues, the actual clinical value of measurements of ROS is less clear (Table 11.3). ROS reacts with DNA as the primary target to produce various byproducts such as 8-hydroxydeoxyguanosine (8-OHdG) (Hwang and Kim 2007). Oxidative stress can also be measured by oxidative modification of proteins, peptides and amino acids, lipids, cell membranes, and small molecules. For example, markers such as nitro-tyrosine, modified albumin, oxidized low density lipoprotein (ox-LDL), malondialdehyde (MDA), and arachidonic acid derived oxidation products (plasma F(2)-isoprostanes) have been used to measure ROS.
Many enzymes, such as superoxide dismutase (SOD), nitric oxide synthase (NOS), myeloperoxidase (MPO), and glutathione peroxidase, process nitric oxide, a key ROS. In addition, small molecule REDOX acceptors also process ROS. These include vitamin C, vitamin E, and uric acid (Pitocco 2013; Sung et al. 2013). Glutathione (GSH) levels are a direct measurement of ROS generation (Motawi et al. 2013). For example, GSH levels decrease in diabetic patients due to increased mitochondrial oxidative activity and the formation of byproducts of NADH dehydrogenase. Oxidative damage of DNA, amino acids, lipids, and small molecules also has unique repair systems such as DNA ligase and methionine sulfoxide reductase (Hwang and Kim 2007). Lipoprotein-associated phospholipase A2 (Lp-PLA2) hydrolyzes oxidized phospholipids to generate lysophosphatidylcholine and oxidized fatty acids, and Lp-PLA2 activates the innate immune system.
Oxidative repair and formation is in flux, and clinical correlations often use several biomarker measurements to account for overall oxidative stress (Sung et al. 2013). Oxidative stress is further complicated by the amplification caused by pro-inflammatory cytokines released by immune cells and stimulated by bacterial lipopolysaccharides (LPS) during infections. Therefore, many biomarkers, including those for immune cell activation, are accounted for in clinical assessments. Urinary biomarkers of oxidative damage has been an attractive topic of research due to the noninvasive sampling process involved (Il’yasova et al. 2012). Thus, the range and depth of biomarkers tested is broad and wide, and correlations vary greatly between the actual species measured. Of the biomarkers tested to date, 8-OHdG, ox LDL, and MDA are among the most clinically verified (See Table 11.3).
Table 11.3
Markers of oxidative stress
Measurement | Markers | Key cellular pathways |
---|---|---|
DNA damage | 8-OHdG | NOS |
DNA ligase | ||
SOD | ||
NOX | ||
NADH/GSH | ||
Anti-oxidants (diet) | ||
Innate immune cells | ||
Lipid oxidation | ox LDL, MDA | Lipase |
Innate immune cells |
In obesity and metabolic syndrome, rapid weight loss correlates with reduced oxidative stress as measured by 8-OHdG, oxLDL, and MDA. Oxidative stress measured by these three biomarkers significantly increases (P < 0.05) with fast blood glucose and HbA1c in type 1 diabetics (Goodarzi et al. 2010; Holvoet et al. 1998). Statistically significant higher values of ROS markers were observed in diabetic patients along with slight reduction in the synthesis of nitric oxide and a decrease in the antioxidant levels (Ramakrishna and Jailkhani 2007; Al-Rawi 2011). Of the oxidative stress marker measured, markers of DNA damage, 8-OHdG and MDA, appear to be the most clinically sensitive to diabetes. Dieting and improved antioxidant intakes significantly improves the levels of these markers in diabetic subjects, and the improvements correlate with improved fasting plasma glucose value and HbA1c (Armstrong et al. 1996).
Alternative approaches to measure oxidative stress by NO, SOD, GSH, or REDOX acceptors poorly differentiate normal patients from diabetics with hyperglycemia (Motawi et al. 2013). Small decreases in MPO (15 %) and phospholipase activities (Lp-PLA2) (4 %), after adjustment for age and sex, were observed in diabetic patients (Tumova et al. 2013). The levels of plasma F(2)-isoprostanes, ox-LDL, and Lp-PLA2 as markers of oxidative damage were unchanged across the risk categories of metabolic syndrome (Seet et al. 2010). A small decrease of 12 % in oxidized low density lipoprotein (ox-LDL) levels was reported in diabetics with hyperglycemia. Additionally, ox-LDL levels increase in diabetic patients with coronary artery disease (CAD) but do not increase in diabetic patients without CAD, and these levels are impacted by sulfonylureas therapy (Motawi et al. 2013).
Hypoxia and uremic toxins increase oxidative stress during kidney and heart disease. Antioxidant therapies show significant reduction in event risk but have not been shown to improved survival (Sung et al. 2013). Innate immune cell involvement shows a significant increase in oxidative stress and event risk. However, the predictive value of ROS markers is weakly correlated (R ~ 0.4) with diabetes given the complexity of oxidative stress status of the body. Many factors, such as age, diet, and other diseases, contribute to oxidative stress levels. Therefore, biomarker use in a clinical setting is impractical for general diabetic patient care, as all factors must be accounted for (Motawi et al. 2013). Although recent trials on anti-oxidants treatments (like alpha-lipoic acid) in type 2 diabetes have not shown decreases in ROS or AGE levels (Porasuphatana et al. 2012), ROS markers remain a valuable research tool.
11.4 Impact of Obesity
Adipose tissue is now recognized as having an endocrine function that serves as a source of adipokines and as having an innate immune function that serves as a source of cytokine and chemokine signals (Preedy and Hunter 2011). Release of adipokines allows insulin independent glucose uptake and fatty acid oxidation in muscle and liver. Obesity impairs the secretion of adipokines and causes insulinemia, hyperglycemina, lipidemia, and reduced appetite suppression (See Table 11.1). Impaired adipokine secretion helps explain the impact of excess fat storage during glycemia where an impaired AMP-activated protein kinase (AMBK) pathway results in decreased glycolysis and reduced fatty acid oxidation. Obesity and insulin resistance increase the release of free fatty acids from the liver. Lipid loading causes the adipose tissue to become inflamed and hypoxic, and it induces cytokine and chemokine release and stimulation of innate immune cells. However, these new understandings of how adipokines relate to insulin resistance and the resultant hyperglycemia has had limited benefits for today’s diagnosis and treatment.
Lifestyle changes such as exercise and diet for weight loss and glycemic control have not stemmed the tide of the epidemic of obese adults with diabetes. Bariatric surgery has become a common treatment for weight loss and glycemic control in obese adults with diabetes (Maggard-Gibbons 2013), and studies have shown that at the 2-year follow up after bariatric surgery, adiponectin, leptin, insulin resistance, and body mass indexes (BMI) continue to show improvement (Trakhtenbroit et al. 2009; Ballantyne 2005). Glucose metabolism and insulin resistance are improved short term by caloric restriction, and decreased fat mass leads to long-term improvements in insulin resistance and returns adipokines secretion closer to normal levels (Cumb 2005; Sasaki et al. 2014). It is common for bariatric surgery patients to also have non-alcoholic fatty liver disease (NAPLD), and NAPLD progresses to non-alcoholic steatohepatitis (NASH) in morbidly obese subjects. Hepatic insulin resistance and steatosis precede the development of type 2 diabetes. Thus, bariatric surgery improves steatosis, fibrosis, lipidemia, and inflammation markers in NASH and NAPLD patients (Sasaki et al. 2014).
Adipokines have led to several new therapeutic approaches for treatment of obesity and diabetes. Adiponectin administration in rodents is insulin-sensitizing and decreases body weight, but this result is not applied to humans (Haluzik 2005). Thiazolidinedione (TZD) activation of peroxisome proliferator-activated receptors (PPARγ) increases adiponectin and transcription of important regulators in transport, synthesis, storage, and oxidation of fatty acids. Leptin therapy normalizes gonadotrophin secretion and menstrual function but is only used in rare human disorders that cause congenital leptin deficiency and lipodystrophy. Leptin treatment causes reductions in hyperglycemia, hypertriglyceridemia, and hepatic steatosis (Gorden 2003)
Gherlin and peptide tyrosine tyrosine (aka peptide YY, PYY, pancreatic peptide, and YY3-36) are also important factors that impact obesity through appetite. Gherlin and PYY are released by cells in the ileum and colon in response to feeding (Soares et al. 2008; Knudsen et al. 2014). Inhibition of ghrelin impacts growth hormone secretagogue receptor (GHSR) and neuropeptide Y (NPY) pathways and reduce appetite, food intake, body weight, and adiposity. Thus, the gherlin pathway is an attractive therapeutic target. When PYY is injected and enters the central nervous system, it acts as an orexigenic and increases appetite. It also has functions related to digestion and absorption and is associated with both increased and decreased appetite. Obese people secrete less PYY than non-obese people, and studies have shown that it inhibits appetite in rodents and primates. Leptin treatments have also been shown to reduce appetite, but obese people develop a resistance to leptin, so it is not an effective treatment for obesity.
11.5 Adipokine Markers
While adipokine biomarkers are closely associated with obesity and diabetes, the predictive value of these markers has not made the grade for routine diagnostic use (See Table 11.1). Adiponectin, leptin, and gherlin have the strongest correlations and are useful for pathway research (See Chap. 1 and Table 11.4), and levels of these three markers improve after bariatric surgery (Ballantyne 2005; Gumbs et al. 2005). Leptin is directly correlated to patient fat mass and diet/exercise balance (Meiera and Gressner 2004). In addition, leptin and adiponectin correlate with hypertrophic adipose tissue in obesity and reduced glycolysis, reduced fatty acid oxidation, insulin resistance, and type 2 diabetes. An increase in the ratio of adiponectin to leptin improves the correlations (Satoh 2004). Ghrelin levels also generally correlate with obesity. Leptin and ghrelin exert important roles on body weight regulation, eating behavior, and reproduction by acting on the central nervous system and target reproductive organs. The relevance of other adipokines has not been resolved or strongly correlated and are still of question (See Chap. 1).
Table 11.4
Markers of adipose stress
Measurement | Markers | Key cellular pathway |
---|---|---|
Impaired glycolysis and fatty acid oxidation | Adiponectin | AMPK |
PPAR α | ||
Appetite suppression | Gherlin | GHSR |
Neuropeptide Y (NPY) | ||
JAK STAT | ||
Fat mass and energy balance (intake/exercise) | Leptin | GHSR |
Neuropeptide Y (NPY) | ||
JAK STAT |
11.6 Fatty Acid Markers
Obesity increases adipose release of free fatty acids (FFA) and triglycerides (TG), and adipose tissues can produce FFA and reduce TG by the acylation stimulating protein (ASP). Interaction of the ASP precursor (compliment C3), factor B, and adipsin produce ASP. Elevated FFA concentrations are predictive of pre-diabetic progression to diabetes but are not diagnostic of diabetes, and a decrease in fasting FFA adversely impacts glucose-induced insulin release by pancreatic ß-cells. FFA acid uptake and metabolism are required for normal islet function (Lam et al. 2002). Insulin increases the production of ASP. Fatty acid oxidation, PPAR gamma activation, and mitochondrial activity in the muscle and liver are also upregulated by insulin (Turner et al. 2007). Fasting plasma ASP concentration correlates with fasting serum triglyceride during the oral fat tolerance test (OFTT) and negatively correlates with the glucose disposal rate and high-density lipoprotein (HDL) concentrations (Koistinen 2001). However, there is no correlation between fasting plasma ASP concentration and TG in lean healthy men, and fasting plasma ASP concentrations are only mildly elevated in obese and diabetic subjects (Koistinen 2001; Peake et al. 2005). Given these correlations, fatty acid stress is currently best measured by typical and proven diagnostic markers of lipidemia (See Table 11.5). ASP is highly correlated to ketosis-prone diabetes (Liu et al. 2014). Other adipose markers such as lipase, FABP, and adipose factor are involved in fatty acid stress during obesity but have poor diagnostic predictive value for diabetic onset (See Chap. 1).
Table 11.5
Markers of fatty acid stress
Category | Markers | Key cellular pathway |
---|---|---|
Impair fatty acid tolerance | Fatty acids (FFA) | ASP |
Triglycerides (TG) | DCAT | |
Lipids (HDL/LDL) | SREBP, FXR and LXR |
Nuclear receptors such as sterol regulatory binding protein (SREBP), farnesiod X receptor (FXR), and liver X receptor (LXR) are pharmacological targets for improving lipidemia, bile acid production, and glucose metabolism in type 2 diabetes (Ding 2014). Synthetic derivatives of triiodothyronine, retinoic acids, fatty acids, eicosanoids, oxysterols, bile acids, steroids, xenobiotics, mineralocorticoids, glucocorticoids, androgens, estrogens, progesterone, retinoic acids, synthetic estrogens, riccardin, and podocarpic acid amides have all been identified as potent agonists of SREBP, FXR, and LXR (Lui 2005; Makishima 2005). Acyl CoA diacylglycerol transferase (DGAT) is an important enzyme in TG synthesis and another important pharmacological target (Subauste and Burant 2003). Treatment with n-3 fatty acids as inhibitors of DGAT decreases the availability of fatty acids for triglyceride synthesis (Subauste and Burant 2003; DeVita and Pinto 2013). Statins are inhibitors of 3-hydroxy-3-methylglutaryl CoA reductase (HMG) and lower cholesterol. Reduced cholesterol directly reduces DGAT expression by increasing the transcription factor SREBP and upregulates fatty acid oxidizing enzymes and inhibitors (Chen and Farese 2005).
11.7 Inflammation Markers in Diabetes
Adipocytes produce tumor necrosis factor alpha (TNFα), IL-1β, and IL-6 during obesity and are shown to cause insulin resistance (Kern et al. 2001; Lorenzo et al. 2008; Cawthorn and Sethi 2008; Coppack 2001). Adipose tissue also contains a significant amount of stromovascular fraction that is made up of preadipocytes, endothelial cells, smooth muscle cells, fibroblasts, leukocytes, and macrophages which can produce substantially more TNFα than adipocytes (Cawthorn and Sethi 2008). Production of IL-1 and IL-6 is regulated differently from TNFα, and interactions between macrophages and adipocytes control adipocyte differentiation and cytokine expression (Xie et al. 2010; Cawthorn and Sethi 2008). TNFα induces cytokine secretion by inflamed cells and is cytotoxic. IL-6 promotes differentiation of B cells and stimulates antibody secretion by plasma cells. IL-1 stimulates T cells, attracts macrophages and neutrophils, and activates NK cells (Gao et al. 2014). Cell signaling by TNFα, IL-1β, and IL-6 decreases GLUT4 in adipocytes. Both IL-6 and TNF- α inhibit insulin action through the Akt-PIK3 pathway. They also both initiate a host of other cytokines (e.g. IL-8, IL-18β) and chemokines (e.g. MIC-1, MIP-2, MCP-1, CINC-1) to recruit macrophages and other innate immune cells to inflamed tissue. Chemokines direct chemotaxis to distressed cells and cause neutrophils, macrophages, T-lymphocytes, eosinophils, natural killer cells, and other innate immune cells to build-up in the adipose. These pathways can be characterized by several markers (See Table 11.6).
Table 11.6
Inflammatory markers adipose stress
Category | Markers | Key cellular pathway |
---|---|---|
Cytotoxic adipose response in obesity | TNF-α, IL-6, and IL-1 | NFκB activation Akt-PIK3 inhibition |
Prolonged inflammation exposure due to obesity and diabetes | CRP TNFRs | TNF-α IL-1 IL-6 |
Macrophage response | YKL-40, IL-6 , MCP-1 | M2 pro-inflammatory polarization |
During insulin resistance, cytokines and chemokine secretion is elevated in adipose tissue and muscle (Beavers and Nicklas 2011; Cawthorn and Sethi 2008). Adipose tissue produces a five-fold increase in TNFα or IL-1β and a 40-fold increase in IL-6 secretion which correlates with obesity measured by BMI (Kern et al. 2001; Zhao et al. 2014). Plasma values of IL-8, IL-18, and Monocyte chemotactic protein (MCP-1) are elevate to a lesser extent during insulin resistance, and plasma levels of IL-1β, TNFα, and IL-6 also correlate with insulin resistance and adipose dysfunction (Beavers and Nicklas 2011; Kern et al. 2001). However, clinical utility is limited, as values of IL-1β, TNFα, and IL-6 show high intra and inter-individual variability with overlap between healthy and diabetic individuals (Kern et al. 2001; Breslin et al. 2012; Beavers and Nicklas 2011). Nonspecificity is also an issue, as cytokines are also markers of endothelial dysfunction and vascular inflammation (Beavers and Nicklas 2011).
Tumor necrosis factor receptor (TNFR) is released as a soluble form (sTNFR) into plasma during diabetic inflammation (Cawthorn and Sethi 2008), and in animal studies, neutralization of TNFα by sTNFR leads to a significant improvement in insulin resistance (Hotamisligil et al. 1993). However, in both obese and nonobese adults with damaging lipidemia, plasma sTNFR levels increase and are not more correlated to obese adipose than TNFα (Cawthorn and Sethi 2008; Good et al. 2006). Plasma sTNFR has been correlated to progression of chronic kidney disease (CKD) (Krolewski et al. 2012). This chronic response likely reflects prolong TNFα activation and not acute innate immune activity (See Table 11.6). In contrast, lipocalin (LCN-2), aka neutrophil gelatinase-associated lipocalin (NGAL), is secreted by neutrophils during renal ischemia reperfusion injury and is a marker of acute kidney injury (AKI) (Soni et al. 2009). However NGAL is only weakly associated with obesity and diabetes through levels of fasting triglycerides and LPS-binding protein and FFA intake (Moreno-Navarrete et al. 2010)
C-Reactive protein (CRP) synthesis is upregulated by cytokines, predominantly IL-6, and is a precise measure of systemic mild inflammation that returns to baseline 12–24 months after weight loss from bariatric surgery (Gebhart et al. 2014). Mild inflammation as measured by CRP is consistently shown to be an independent predictor of metabolic syndrome, obesity, and diabetes (Seet et al. 2010) (See Table 11.6). CRP activates the complement pathway, binds phosphocholine, and promotes clearance of apoptotic cells (Szalai 2004). CRP levels are significantly increased in obese patients during hyperglycemic and diabetic ketoacidosis crises as compared to obese controls (Stentz et al. 2004), and these CRP values return to normal after insulin treatment and resolution (Stentz et al. 2004; Peuchant 1997). Increased fat mass correlates with CRP (Tsuriya et al. 2011). However, these CRP changes are small in magnitude and cannot predict type 2 diabetes or the prediabetic state but are clearly indicative of prolonged chronic inflammation.
Mild elevations of peripheral white blood cell (WBC), neutrophil, granulocyte, lymphocyte, and monocyte counts are associated with the risk of diabetes (Gkrania-Klotsas et al. 2010; Vuong et al. 2014). However, these innate immune cell levels are not able to differentiate progression to diabetes. In diseased adipose tissue, macrophages are polarized into pro-inflammatory M1 forms by IL-10, CD8 positive T cells, interferon (INF-γ), and immunoglobulins from B cells. Alternatively, macrophages are polarized in healthy adipose tissue into anti-inflammatory M2 forms by regulatory T cells (Tregs), eosinophils, and IL-4. As polarization occurs in tissue, few blood based markers for macrophage responsivity exist today. However, general macrophage markers such as chitinase-3-like protein 1 (YKL-40), which is secreted by macrophages in inflamed tissues and causes activation of the Akt pro-survival signaling (anti-apoptotic pathway), can be used. YKL-40 levels are elevated in patients with vascular disease, hypertension, diabetes, and obesity (Ridker 2014). However, YKL-40 is also elevated in neutrophils. MCP-1 is another example of a marker secreted primarily by macrophages, endothelial cells, and adipocytes (Patel et al. 2013).
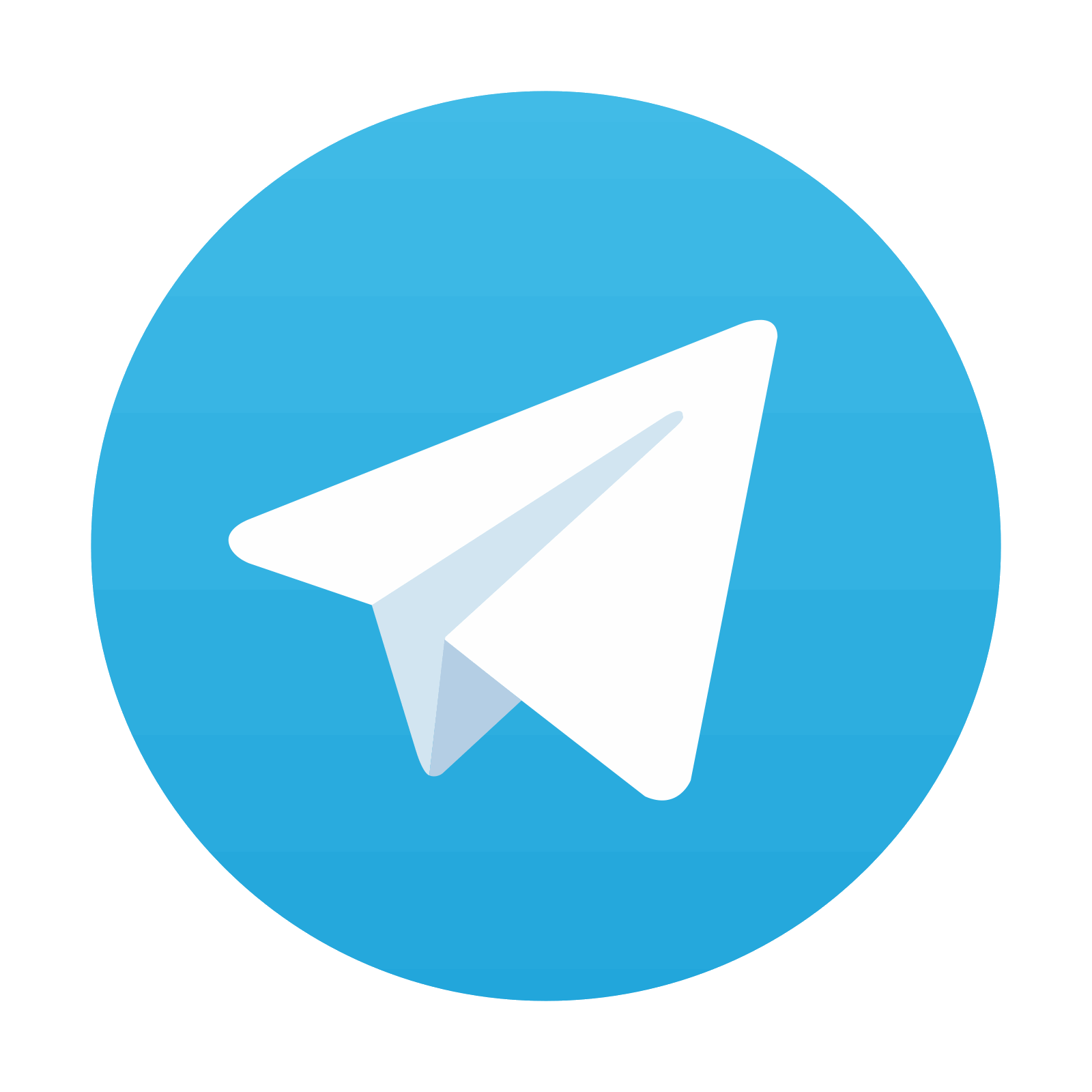
Stay updated, free articles. Join our Telegram channel
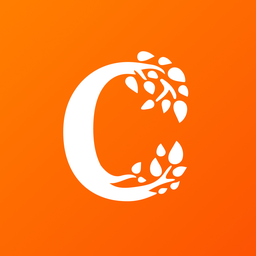
Full access? Get Clinical Tree
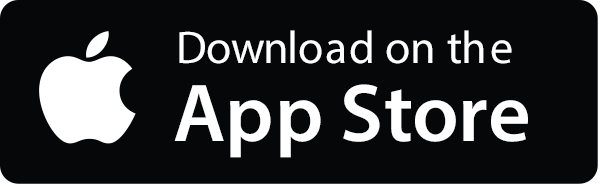
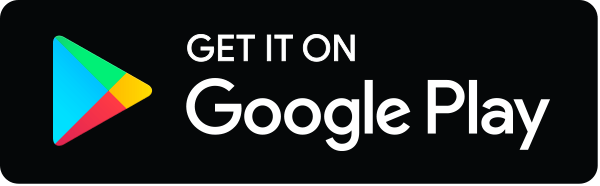