7 Physiological Imaging
The past three decades have seen the implementation and widespread clinical use of noninvasive anatomic and physiological magnetic resonance imaging (MRI) methods in the field of neuroimaging of brain tumors. Along with advances in neuroimaging, tremendous progress has been made in other fields of neuro-oncology such as stereotactic and navigational neurosurgical techniques, intraoperative cortical and subcortical stimulation mapping methods, immunohistochemical methodologies, and a variety of conformal radiation therapies such as gamma knife radiosurgery and cyberknife radiation therapy. More recently, the unraveling of molecular biology, of the origin of neural stem cells, and of the cancer genetics of brain tumors has led to an entirely new era of exploring the pathogenesis and therapeutic targeting of brain tumors.
Neuroimaging plays a critical role in the diagnosis and preoperative planning of brain tumor cases and also serves as a means for evaluation during or after therapy. Recent advances in technology have fostered the development and clinical application of several new physiology–based MRI methods that provide information not readily available from traditional anatomic imaging. Combined anatomic and physiological MRI promises more comprehensive characterization of tumor and a better understanding of tumor biology. Recent developments and clinical trials of highly selective chemotherapeutic agents, such as those that target specific cancer signaling pathways, tumor-produced growth factors, or angiogenic tumor vessels, promise much hope and reinforce the conviction of every practitioner in the field of neuro-oncology that growth control, albeit not cure, of malignant brain tumors is potentially within reach in the next decade.
Controversy
• Despite their potentially powerful role in advancing knowledge of brain tumor biology, physiology–based MRI methods still await much–needed validation and correlation with clinical outcomes data.
Brain Tumor Imaging
Computed tomography (CT) of the brain is very sensitive in detecting acute hemorrhage, hydrocephalus, and herniation, and can be helpful in detecting areas of blood–brain barrier and defining the contrast–enhancing tumor border. However, CT is primarily reserved as an initial screening method to exclude a potentially life–threatening intracranial process, and it suffers from several important limitations. First, CT is not optimally suited for detecting subtle changes in brain parenchyma, such as nonenhancing tumor or infiltrative changes, due to its intrinsically low soft tissue contrast.1 Computed tomography findings can be rather subtle in cases of infiltrating tumor and easily overlooked. Second, CT does not provide flexible multiplanar acquisition, thus limiting the three–dimensional depiction of tumor. Third, CT involves ionizing radiation, and its iodinated contrast agent can cause serious allergic reaction. Finally, CT is largely confined to providing anatomic information. Even with the addition of intravenous contrast agent, CT is still inferior to MRI in terms of soft tissue resolution, multiplanar capability, and physiology–based applications.
Contrast–enhanced MRI provides exquisite anatomic detail, has multiplanar capability, and does not require ionizing radiation; therefore, it is the current imaging standard for brain tumor diagnosis and therapy monitoring. In clinical practice, the most widely accepted standard imaging protocol includes at least the following two sequences: contrast–enhanced T1–weighted imaging and fluid–attenuated inversion recovery (FLAIR) imaging.
Unfortunately, anatomic MRI is largely limited to depicting morphological abnormality and suffers from nonspecificity. Different disease processes can appear similar or a single disease entity may have varied imaging findings. The underlying metabolic or functional integrity of the brain cannot be adequately evaluated based on anatomic MRI alone. To that end, several physiology–based MRI methods have been developed and have become part of an armamentarium of imaging paradigm to improve tumor characterization. It should be noted that most of these advanced MRI methods are still considered investigational and require further clinical validation and outcomes data to determine their role in clinical management of patients.
Physiology–Based Magnetic Resonance Imaging
Diffusion–Weighted Magnetic Resonance Imaging
Diffusion of water in biological systems, particularly within the brain, is affected not only by the complex interaction between the intra–and extracellular compartments but also by the cytoarchitecture of the microstructures and permeability barriers. Diffusion of water molecules through the magnetic field gradient produces intravoxel dephasing and a loss of signal intensity. Because this microscopic diffusional motion is so small, a large gradient strength or duration is needed to produce observable signal loss from diffusion. By utilizing bipolar pulsed gradient methods, microscopic diffusional motion is detected by a change in the magnitude of moving spins due to phase dispersion. To detect this highly sensitive motion, an ultrafast imaging method, such as the echo planar technique, is needed to acquire a sufficient number of images in the range of milliseconds to produce meaningful information.
Apparent diffusion coefficient (ADC) characterizes the rate of diffusional motion (given in millimeters squared per second). The ADC takes into consideration the heterogeneous environment of brain cytoarchitecture and factors other than diffusion, such as temperature, perfusion, and metabolic rates, that can affect the measurement of microscopic thermal motion. High ADC implies relatively unrestricted water motion. Low ADC indicates restricted diffusional motion, as seen in acute cerebral ischemia. The diffusion sensitivity parameter, b value, is related to duration, strength, and time interval between the diffusion–sensitizing gradients. A typical b value used in clinical imaging is in the range of 900 to 1,000 seconds/mm2. The higher the b value, the more sensitive the diffusion imaging is in obtaining greater contrast and detecting areas of restricted water motion.
Anisotropic diffusion is defined as having different diffusional motion in different directions, as is the case in normal myelinated white matter tracts in the brain. Diffusion of water molecules is far less restricted along the parallel plane of the axonal fibers than in perpendicular direction. White matter anisotropy can be demonstrated by comparing diffusion–weighted images with bipolar gradients placed in three orthogonal directions in which hyperintensity in the white matter is visible when the diffusion encoding is perpendicular to the fiber orientation. By combining the information from the three orthogonal data, an orientation–independent image is created without the artifact from normal white matter anisotropy.
Pitfall
• Physiological imaging methods based on the echo planar imaging technique are prone to susceptibility artifact and geometric distortion. The susceptibility artifact is most pronounced at the brain–bone–air interface such as the anterior and middle cranial fossa or cerebellar hemisphere near the petrous apex.
Echo planar imaging (EPI) is currently the most widely used MRI technique for clinical application of diffusion–weighted imaging for the diagnosis of acute stroke and other brain disorders such as abscess, epidermoid cyst, traumatic shearing injury, or necrotic encephalitis. It is the fastest available MRI method, which allows the entire set of echoes needed to form an image to be collected within a single acquisition period of 25 to 100 ms.2 The data are obtained by forming a train of gradient echoes by repeated reversal of a large gradient capable of very rapid polarity inversion to complete k–space filling after a single radiofrequency (RF) pulse. Each gradient echo is phase encoded separately by a very brief blipped gradient or a weak constant phase–encoding gradient. Although the long echo train renders the images sensitive to chemical shift and magnetic susceptibility artifacts, EPI virtually eliminates motion artifact. The chemical shift artifact is overcome by routine use of lipid suppression, whereas the magnetic susceptibility artifact is manifested prominently at air–bone–tissue interfaces such as those at the skull base, paranasal sinuses, orbits, and petrous temporal bone.3–5
Clinical Application of Diffusion–Weighted Magnetic Resonance Imaging
Diffusion–weighted MRI is now considered the standard imaging method to diagnosis early cerebral ischemia. With the onset of ischemia, there is breakdown of the cell membrane. There is failure of Na2+–K+–adenosine triphosphatase (ATPase) pumps, resulting in an influx of Na2+ into the cell and subsequent cell swelling. At this time the cells are still viable; however, the extracellular space is compressed and restricted in mobility secondary to expansion of the intracellular compartment. This impaired diffusion within the areas of ischemia is due to cytotoxic edema and microstructural changes within the damaged cells. Localized hyperintensities appear on diffusion–weighted EPI images within minutes of the onset of cerebral ischemia well before conventional MRI shows any changes. These rapidly appearing diffusion abnormalities and the decrease in ADC represent the failure of the energy–driven membrane pumps and the subsequent changes in membrane permeability and regional cytoarchitecture restricting water motion. Changes seen on conventional MRI, including the FLAIR sequence, are delayed until there is a breakdown of the blood–brain barrier, which occurs after the membrane pump failure. Ischemia–induced diffusion abnormality may persist up to 14 days following the acute event, and it precedes later–occurring, permanent structural alterations signifying complete infarction. The abnormality on diffusion–weighted MRI and ADC maps returns to baseline approximately 7 to 14 days following the acute event. The sensitivity and specificity of diffusion imaging in the diagnosis of acute infarct exceeds 95% and is far superior to conventional MRI and CT examinations.
In addition to early diagnosis of cerebral ischemia, diffusion–weighted MRI is extremely sensitive in detecting other intracranial disease processes, including cerebral abscess, epidermoid cyst, traumatic shearing injury, toxic or infectious encephalitis, and immediate postoperative brain injury. The exact mechanism of diffusion restriction in each of these disease entities remains not entirely certain, but diffusion–weighted MRI can be extremely helpful in making the diagnosis. Cerebral abscess, for example, can appear indistinct from a cystic brain tumor, and preoperative diagnosis is critical for proper surgical and medical management. Diffusion–weighted MRI demonstrates profound restricted–diffusion abnormality in cerebral abscess (Fig. 7.1), most likely attributable to the increased viscosity of the pus fluid. Similarly, an epidermoid cyst contains highly viscous material, which can explain the characteristic restricted diffusion associated with this entity as shown in (Fig. 7.2). Diffuse axonal injury following trauma represents areas of permanent brain injury due to the rotational shearing force that results in destruction of cytoarchitecture of the affected brain. In toxic or infectious encephalitis, herpes being the prototype, there is widespread necrotizing tissue destruction due to direct injury to the neurons and oligodendrocytes. Again, the end result is altered membrane integrity and shift of water into the intracellular compartment and corresponding restriction of extracellular space and water motion.
Diffusion–weighted MRI can be extremely valuable in accurate interpretation of new abnormal contrast enhancement that develops soon after tumor resection. Fig. 7.3 depicts an area of reduced diffusion on the immediate postoperative MRI following resection of a glioma. On 1-month follow–up MRI, there is a clear focus of abnormal contrast enhancement along the resection margin corresponding to the area of the reduced diffusion on the postoperative MRI. This new enhancement, which can be easily misinterpreted as recurrent tumor, represents an area of brain injury with subsequent blood–brain barrier disruption rather than recurrent tumor. A study shows that this enhancement within the area of diffusion abnormality invariably evolves into a gliotic cavity, as one would expect in any permanently injured brain, and does not represent tumor recurrence.6 Therefore, it is imperative to evaluate any new enhancement within the first few months after surgery in the context of diffusion abnormality on the immediate postoperative MRI following tumor resection.
Pearl
• Diffusion–weighted MRI can be extremely valuable in accurate interpretation of new abnormal contrast enhancement that develops soon after tumor resection.
Diffusion tensor imaging is the latest application of diffusion–weighted MRI, where white matter integrity can be depicted on a three–dimensional map.7 Although still in their clinical infancy, diffusion tensor imaging and tractography are promising, noninvasive tools to study the white matter tracts and are likely to become an important and integral part of preoperative planning of brain tumors in the future.
One of the major pitfalls of diffusion–weighted imaging is related to the technique’s intrinsic sensitivity to lesions containing high magnetic susceptibility, such as blood products, calcium or metal, and bone or air. The susceptibility artifact caused by the paramagnetic or ferromagnetic material can cause spurious signal changes on an MRI that simulate pathological processes such as infarct or abscess, and hence the interpretation of diffusion–weighted images must be done by concomitant review of anatomic MRI. This is particularly true in the immediate postoperative state, in which there is usually a combination of blood products and surgical material within the surgical bed that can cause prominent susceptibility artifacts on diffusion–weighted imaging. As demonstrated in Fig. 7.4, an intracranial parenchymal hematoma can show apparent reduced diffusion on diffusion–weighted imaging due to susceptibility effect caused by subacute and chronic blood products, methemoglobin, and hemosiderin.
Fig. 7.1a–c A 41–year–old man with right frontal pyogenic cerebral abscess. (a) Axial fluid–attenuated inversion recovery image demonstrates a large mass into the corpus callosum (arrow). (b) Axial postcontrast T1–weighted magnetic resonance image shows a large rim–enhancing, centrally necrotic mass (arrowheads) within the right frontal lobe, suggestive of an aggressive neoplasm. (c) Axial diffusion–weighted image clearly demonstrates marked reduced diffusion (arrows) within the mass consistent with a pyogenic abscess, which was confirmed in surgery.
Pitfall
• Para– or ferromagnetic materials such as blood products or calcium within the brain can simulate pathology on diffusion–weighted and perfusion MRI.
Dynamic Contrast–Enhanced Perfusion Magnetic Resonance Imaging
Dynamic contrast–enhanced perfusion MRI of the brain provides hemodynamic information that complements the anatomic information attainable with conventional MRI. Contrast–enhanced perfusion MRI methods exploit signal changes that accompany the passage of a paramagnetic contrast agent through the cerebrovascular system and can be used to derive information on blood volume and flow.8–10
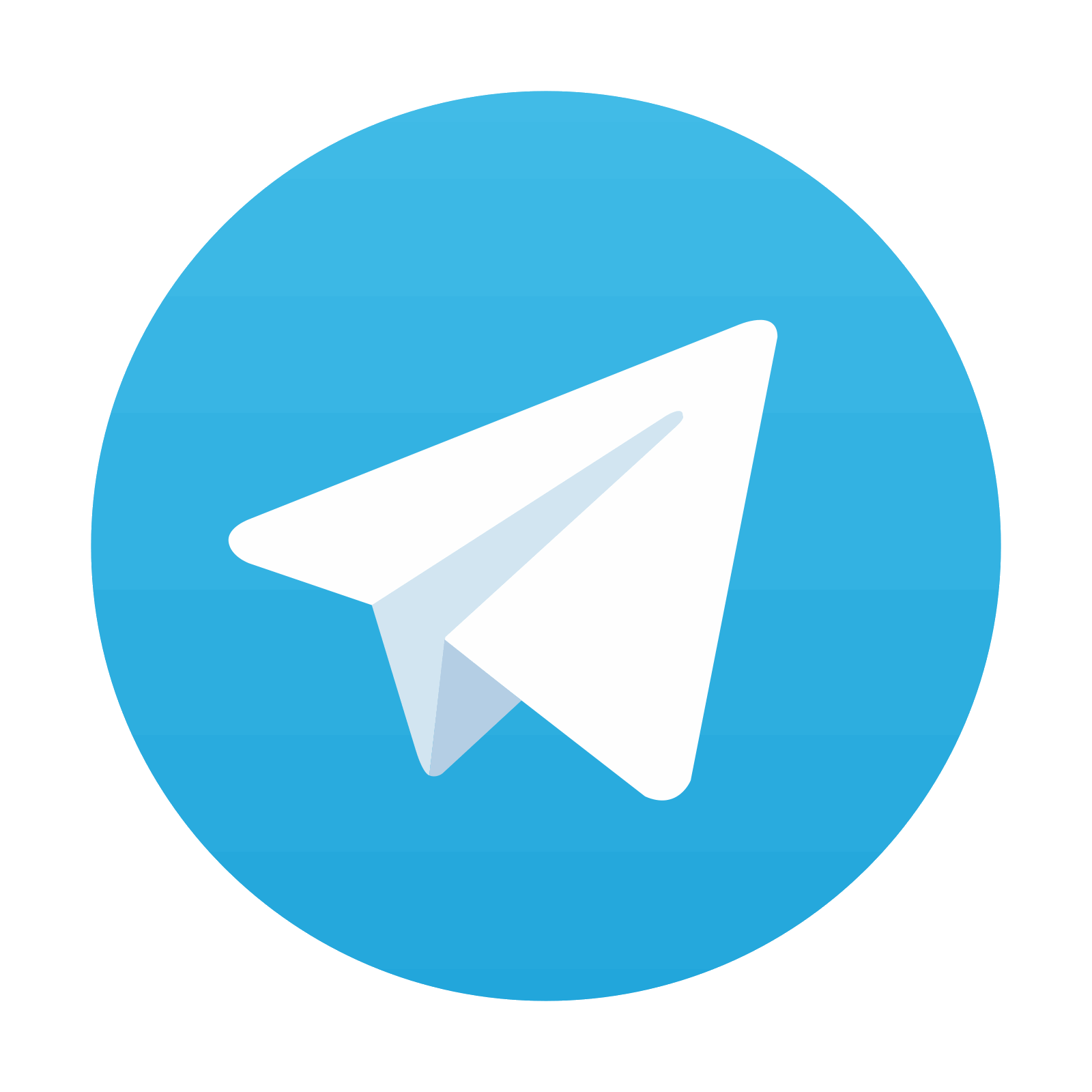
Stay updated, free articles. Join our Telegram channel
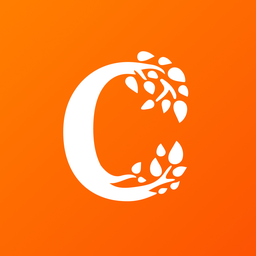
Full access? Get Clinical Tree
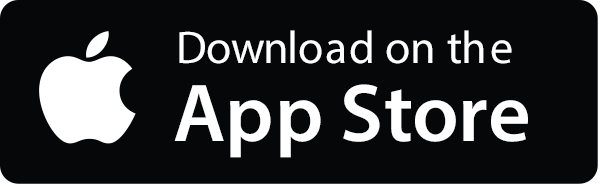
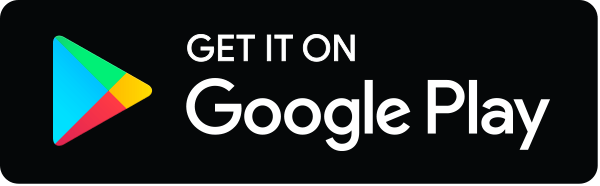