Chapter Outline
PRINCIPLES OF RADIATION ONCOLOGY
THE PHYSICAL BASIS OF RADIOTHERAPY
TECHNOLOGICAL ADVANCES IN RADIATION ONCOLOGY
CLINICAL TREATMENT WITH RADIATION
Principles of Radiation Oncology
Radiation oncology is an effective and often critical component of multidisciplinary cancer care for many childhood malignancies. The delivery of radiation for the pediatric population is highly complex because of the diversity of patients, tumor location, and biology. Additional concerns regarding the use of radiation for this patient population include a high likelihood of cure, coupled with the well-known and often lifelong complications from oncologic treatment. As survival from childhood cancer has improved, there is better recognition of the impact that long-term toxicities can have on the quality of life for patients. Practitioners have also learned a great deal over the past few decades regarding the required dose of radiation to provide cure, as well as the dose that specific healthy organs can tolerate. For each radiation plan it is now easier to deliver the desired target dose while minimizing the dose to healthy organs, allowing for disease control with decreased morbidity. Still, radiation is not without side effects and for each patient the risks and benefits must be considered to determine an acceptable treatment plan. Multimodality treatment is required for nearly all pediatric cancer patients, and physicians from different subspecialties must work closely together to determine the best management for these children. The desires of children and their parents must be taken into consideration to determine what life-long side effects are considered acceptable in order to achieve the best outcome.
The Physical Basis of Radiotherapy
Radiation is by no means a novel phenomenon; background radiation is ever-present. The understanding and use of radiation, however, is a relatively recent development in human history. The discoveries of x-rays in 1895 by the German physicist Wilhelm Konrad Röntgen and radioactivity in 1898 by Henri Becquerel led to the recognition of biologic effects of radiation. By the early part of the twentieth century, ionizing radiation had rapidly come into use to treat malignant and benign conditions. At the 1922 International Congress of Oncology in Paris, Coutard presented the first evidence of the use of fractionated radiotherapy to cure advanced laryngeal cancer without disastrous sequelae. This event marked the beginning of the field of radiation oncology.
Ionizing radiation, which includes both particulate radiation and electromagnetic waves, produces ionizations and excitations during the absorption of energy in exposed tissue. Electromagnetic waves are part of a broad spectrum that includes radio waves, microwaves, visible light, x-rays, and gamma rays. In therapeutic radiation oncology, x-rays, gamma rays, and particulate radiation are used. Gamma rays and x-rays share similar general properties, differing in the source and energies. X-rays are produced when charged particles, generally electrons, are accelerated and bombard a high-density target. The target, most commonly tungsten, emits photons of varying energies up to the peak energy of the accelerated electrons. The energy (E) of the photons is determined using the relationship E = hv, where h is the constant of proportionality known as the Planck constant , and v is the frequency of the wave. Substituting for the frequency, the equation becomes E = hc/λ, where c is the speed of light and λ is the wavelength. In contrast, gamma rays are emitted by a source; the most commonly used source for gamma rays used in therapeutic radiation is cobalt.
Radiation may be directly ionizing or indirectly ionizing. Direct ionization results in a direct disruption of the atomic or molecular structure of tissue through which the beam of radiation passes. This disruption causes biochemical and molecular damage. Particle beams are directly ionizing. Electromagnetic waves and neutrons are indirectly ionizing; when absorbed in tissue they give up their energy by producing fast-moving charged particles. These charged particles directly damage tissue. Photons in tissue interact in several different ways, including producing the Compton effect or the photoelectric effect, coherent scattering, pair production, and photodisintegration. The dominant reaction, the Compton effect, is governed by the energy of the photons. This process, shown in Figure 48-1 , involves the interaction of the photon with a loosely bound orbital electron. Part of the energy of the incident photon is transferred to the electron as kinetic energy; this Compton electron may then interact with electrons in the surrounding tissue. The remaining energy is carried away by another photon that is less energetic than the original photon. The probability of Compton interactions is independent of the atomic number of the target tissue.
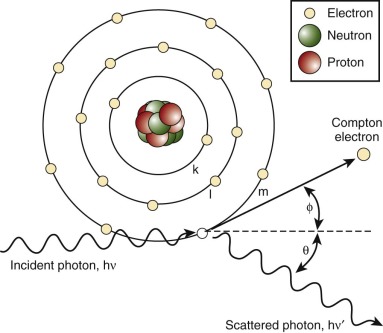
Whereas photons interact mainly with orbital electrons, protons, neutrons, and other heavy particles interact with the nuclei of atoms. Heavy particles also cause showers of lower energy and densely ionizing protons, neutrons, and other particles, depositing large amounts of energy over a very short distance. This is referred to as linear energy transfer (LET) and depends on the type of radiation used. Photons and electrons both have low rates of energy transfer. Heavy particles tend to deposit their energy in a track over a relatively short distance, and these particles are considered high-LET radiation.
External-beam radiation treatment systems produce ionizing radiation by radioactive decay of a nuclide, most commonly cobalt-60, or electronically through the acceleration of electrons or other charged particles such as protons. In a linear accelerator, electrons are accelerated down a waveguide by the use of alternating microwave fields. In a cobalt-60 unit the source is always on, because radioactive decay occurs continuously. The source is moved to an unshielded position to initiate a treatment. In a linear accelerator, no source is present until the unit is energized; there is an on switch and an off switch. The energy spectra are different for the two types of treatment units. Cobalt-60 units emit two monoenergetic gamma rays with each decay, producing a discrete spectrum with peaks at 1.17 and 1.33 MeV. A linear accelerator produces a continuous x-ray spectrum with a maximum energy of E max (the accelerating potential) and all other photon energies down to zero. An average x-ray produces the energy of approximately one-third E max . A linear accelerator can produce a treatment beam of electrons as well as photons. The accelerated electron beam exits the treatment unit under controlled conditions of scatter.
The basic components of the treatment machine include a radiation source, a collimating system to form and direct the beam, inherent shielding for protection, a light field to delineate visibly the area being treated, a control system to turn the beam on and off, a system to rotate the beam, and a support apparatus for the patient. Most treatment machines are assembled with isocentric geometry. The isocenter is a point in space at which all of the treatment machine’s rotational axes intersect. Any mechanical rotation occurs around an axis that passes through the isocenter.
The linear accelerator has many degrees of geometric movement and can produce a treatment field in almost any orientation relative to the patient. Figure 48-2 shows a photon linear accelerator. The beam source is mounted on a gantry that rotates fully about the patient. The patient is on a treatment couch that can pivot with respect to the plane of gantry rotation. This degree of movement allows multiple fields to approach the target volume. In addition, the collimation assembly mounted on the gantry allows the radiation treatment field to be geometrically conformed to the target volume intersection. The delivery of proton therapy has evolved to utilize a gantry system adapted from linear accelerators and cobalt delivery systems.
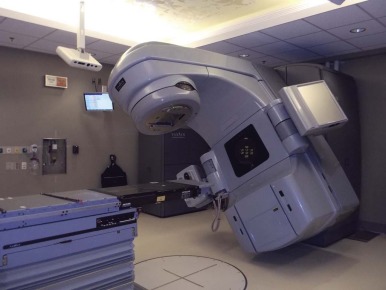
The amount of energy deposited per unit mass represents the absorbed dose of radiation. The official unit of dose is now the gray, in honor of L.H. Gray, the noted British radiobiologist who discovered the oxygen effect; it replaces the older unit, rad. One joule per kilogram is called a gray (Gy) and 1 gray equals 100 cGy. Clinical radiotherapy uses external radiation beam delivery, most often x-rays (photons) or electrons. Linear accelerators accelerate electrons to a given energy, generally 4 to 25 MeV; the electrons bombard a target, usually tungsten, resulting in the formation of an x-ray beam. The x-ray beams contain photons of varying energies up to the peak of the accelerated electrons.
In addition to external-beam radiotherapy, other radiotherapy delivery modalities are used in specific circumstances. Implanting radioactive sources directly into a tumor is known as brachytherapy, from the Greek brachys , meaning short. Brachytherapy can involve the placement of encapsulated sources into a body cavity (intracavitary), directly into the tumor or adjacent tissue (interstitial implants), or on the surface adjacent to the tumor (plaque therapy). Permanent implants in the form of radioactive seeds are placed under the guidance of ultrasound, computed tomography (CT), or magnetic resonance imaging (MRI). Temporary implants are performed by using hollow catheters or loading devices inserted at the time of surgery and then subsequently loaded with radioactive sources. The chief advantage of this technique is to deliver a high dose to the target tissue while sparing the nearby normal tissue. Iridium-192 and iodine-125 are the most commonly used radionuclides. Radionuclides are embedded in seeds and sealed in thin plastic strands that can be inserted into afterloading catheters. Iodine-125, with a relatively short half-life of 57 days, is often used in permanent implants. The other feature of iodine-125 that makes it appealing for use in pediatrics is the lower energy of the photons emitted, which simplify the radiation safety concerns compared with iridium-192. There are radiobiological differences between brachytherapy and external-beam radiotherapy. Implants deliver continuous doses of 30 to 100 cGy per hour to the target tissue over the duration of the implant. This is in contrast to external-beam treatment in which the target tissue receives a short pulse of approximately 200 cGy daily, at a dose rate of 100 cGy per minute, over many weeks. Decreasing the dose rate may reduce the cell kill rate of a given dose, because repair of sublethal damage continues throughout the protracted exposure. High-dose-rate brachytherapy techniques are becoming more widely used, although late complications increase with higher dose rates. For dose rates exceeding 1 Gy per hour, a reduction in the total dose is considered. In addition, fractionation of high dose rates can help to diminish the late complications of high-dose-rate delivery.
Radiobiology
Mechanisms of Radiation Damage
The biologic effects of ionizing radiation result primarily from the formation of double-strand breaks in cellular deoxyribonucleic acid (DNA). Although most single-strand breaks in DNA caused by radiation are repaired, double-strand breaks can result in irreparable damage that leads to mitotic cell death. Photon radiation (x-rays or gamma rays) can cause damage via direct interaction with the DNA molecule or via the formation of free radicals that subsequently damage the DNA (indirect damage). Charged particles such as helium, carbon, and neon cause damage predominantly through direct interactions. High-energy neutrons interact with the nucleus of an atom, resulting in the creation of densely ionizing recoil protons, alpha particles, and nuclear fragments. LET measures the average energy deposited in tissue per unit distance traveled by a particle or photon. Conventional radiation is sparsely ionizing (low LET), whereas fast neutrons and heavy particles are more densely ionizing (high LET).
Radiation causes complex cascades of molecular events that affect cell cycle checkpoints, apoptosis, DNA damage response, and DNA repair. These effects offer many potential approaches to enhancing radiation damage of tumor cells and to protecting normal tissues. These techniques are discussed in this chapter.
Clonogenic Survival Curves
A typical clonogenic survival curve is shown in Figure 48-3 . The surviving fraction is plotted on a logarithmic scale, and the dose of radiation is drawn on a linear scale. The resulting cell survival curve has two distinct regions. In the initial “shoulder” portion of the curve, the fraction of cells killed increases slightly with increasing radiation doses. In the latter part of the curve, killing becomes exponential, which means that a given dose increment kills a constant fraction of cells. The slope of the exponential portion of the survival curve is called the D 0 . This term is related to the inherent radiosensitivity of the cell. The smaller the D 0 , the more sensitive the cells are to radiation. This is in contrast to radio responsiveness, which refers only to the rate of disappearance of a tumor after irradiation and has no correlation with the innate sensitivity of the cells. The D 0 for mammalian cells in vitro generally falls between 1 and 2 Gy. The relatively narrow range of values for widely varying types of cells is striking. The initial nonexponential portion of the curve is believed to result from the capacity of most mammalian cells to repair nonlethal radiation injury. Saturation of the repair capability is thought to occur at the point where killing cells becomes exponential.
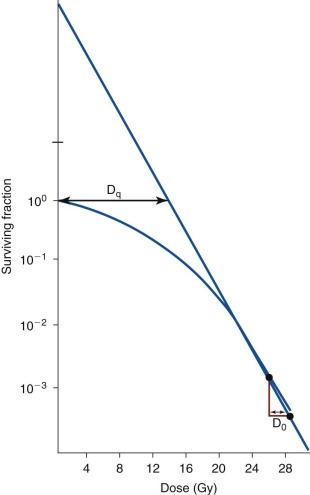
Additional mathematical models have been used to characterize the cellular response to ionizing radiation. One of the most commonly used models, the linear-quadratic survival model, is routinely used to investigate how different tumor repopulation kinetics between radiation treatments influence the scheduling of radiation treatment. As various fractionation schemes have been examined clinically, including hyperfractionation, accelerated fractionation, and combined modality therapy (chemoradiation), the linear-quadratic model has been used to calculate the tolerability of specific tissues to various radiation schedules. The concept of the biologically effective dose, a tool offered by the linear-quadratic model, allows quantification of the effects of radiation schedules on tumors and on normal structures. Although this model has been helpful when applying alternative fractionation schemes to patient treatment, caution must be exercised in its application, because features such as faster tumor growth rate and higher repair capacity result in greater variations in the outcome of the survival fraction. Gaps in treatment, planned or unplanned, also accentuate the differences in the survival fraction under varying growth dynamics. These limitations notwithstanding, mathematical modeling of the cellular response to radiation has contributed greatly to the clinical application of radiation to combating malignancies.
The Oxygen Effect
Tissue oxygenation influences the response of tumors and normal tissues to ionizing radiation. Poorly oxygenated tissues are two to three times more resistant to radiation than are normally oxygenated tissues. Oxygen is thought to mediate indirect damage, combining with free radicals to make DNA damage irreversible. The effect of oxygenation is measured by the oxygen-enhancement ratio. This is the ratio of doses under hypoxic versus aerated conditions required to produce a given level of cell kill. As LET increases, the effect of oxygen enhancement decreases.
Radiation Interactions with Chemotherapeutic Agents
A number of chemical substances may alter the radiation response of a cell. The effects may be additive, such as those of most alkylating agents and antimetabolites, or they may be synergistic, such as those of the antibiotic dactinomycin. Doxorubicin and dactinomycin markedly reduce the shoulder region of the radiation cell–survival curve. Dactinomycin also steepens the slope of the exponential portion of the cell-survival curve and potentiates the radiation effect; thus it is thought to be a true radiation sensitizer.
Combining radiation and chemotherapy can achieve better clinical outcomes than either modality alone but can also potentiate toxicities to normal tissues. Interactions between chemotherapy and radiation can increase the risk for toxicity in normal tissues, even when the two modalities are temporally spaced. In 1959 Dr. G.J. D’Angio described an interesting yet enigmatic phenomenon termed radiation recall. Radiation recall describes the remembering, or reemergence, of an inflammatory reaction that occurs within the radiation field and is prompted by the administration of particular agents days to years after initial radiation exposure. In the original case described by D’Angio, dermatitis emerged within the radiation field after application of actinomycin D to the skin. Although radiation recall is most commonly documented as skin reactions, other tissues and organs are also susceptible to this phenomenon, including oral and intestinal mucosa, larynx, lungs, muscles, and the central nervous system (CNS). The cause of radiation recall remains poorly understood. Although timing and dosing factors clearly impact the risk and severity of the recall reaction, there is no clear radiation dose threshold.
Technological Advances in Radiation Oncology
All technological advances in radiation oncology allow for improved dose delivery to the target or tumor and/or better avoidance of healthy tissues outside of the area at risk of disease recurrence. The field of radiation has benefited from many major developments over the past several decades in the fields of diagnostic radiation and physics, allowing for markedly improved radiation delivery to pediatric cancer patients.
The advent of three-dimensional (3D) imaging with the invention of the CT scanner in 1971 opened a new world for not only diagnostic imaging but also for radiation treatment planning. The ability to define structures and tumors in 3D and to place radiation fields and beam angles with this enhanced information marked a major advancement for the field. Additional imaging developments including MRI and positron emission tomography (PET) further enhanced capabilities to define the area at risk for tumor recurrence. Most radiation centers obtain CT scans in the radiation oncology department in the desired treatment position and employ fusion software to fuse MRI and PET scans to assist in target delineation. Figures 48-4 and 48-5 show fusions used for radiation planning. By defining this region more accurately, practitioners not only improve disease control but can also decrease the volume of healthy tissue receiving unnecessary radiation. Before the use of CT, radiation oncologists used x-rays to plan fields, and beam arrangements were less well guided by anatomic detail. Because of the inability to define tumors as precisely, fields of radiation were large for fear of local recurrence. In addition, the inability to evaluate radiation plans in 3D made the details of dose deposition less clear. Without 3D imaging radiation oncologists would not have been capable of developing the many advanced treatment delivery techniques such as intensity modulated radiotherapy (IMRT) and stereotactic radiotherapy (SRT) that are widely available today.
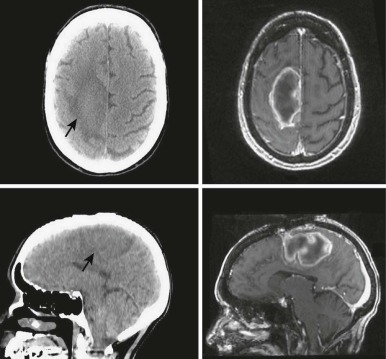
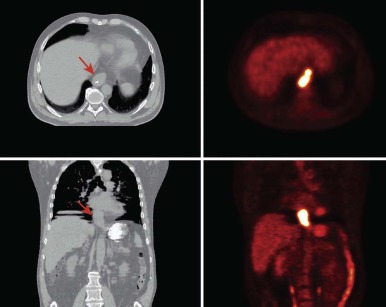
Conformal radiotherapy describes treatment that delivers a high-dose volume that is shaped to conform to the target volume while minimizing the dose to critical normal tissues in the adjacent area. Because conformal radiotherapy attempts to conform the dose to the target, careful and accurate delineation of the target is critical. Patient immobilization for setup accuracy and to limit patient motion is imperative. The International Commission on Radiological Units and Measurements-50 defined target volumes that are used in treatment planning. The gross tumor volume (GTV) is the volume of macroscopic tumor that is visualized on imaging studies. The clinical target volume (CTV) is the volume that should be treated to high dose, typically incorporating both the GTV and the surrounding tissues assumed to be at risk because of microscopic spread of the disease. The planning target volume (PTV) is the volume that should be treated to ensure that the CTV is appropriately covered; this takes into account systematic and random setup errors between treatments and during treatment ( Fig. 48-6 ). The treated volume is the volume of tissue enclosed by a specific isodose line. The treated volume is greater than the PTV.
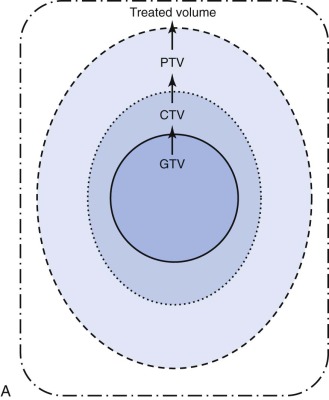
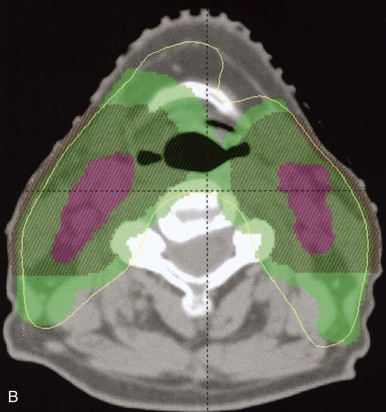
Intensity-Modulated Radiotherapy
Sophisticated planning techniques have significantly improved the ability to tailor the radiation field to the desired treatment region. IMRT is one such novel technique that allows the radiation oncologist to modulate the intensities of individual beamlets within each beam. IMRT requires highly sophisticated software that allows physicians to indicate the desired doses to tumor volumes and organs. This software then runs multiple iterations of treatment plans involving numerous small fields and several beam angles. The physician either accepts the optimized plan or provides new parameters until the desired dose distribution has been met. IMRT accomplishes excellent conformity to target volumes while decreasing the high dose to nearby critical structures. Figure 48-7 demonstrates an IMRT field arrangement and plan for a young child with a localized ependymoma.
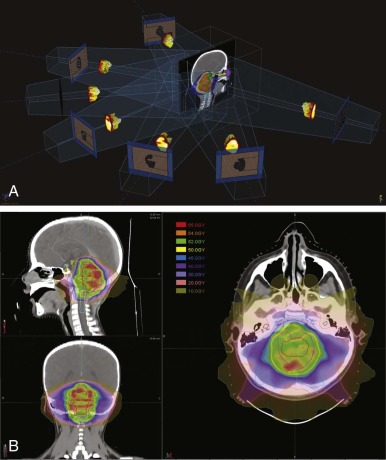
Stereotactic Radiotherapy
Stereotactic radiosurgery (SRS) is delivery of radiation to a target in a single treatment. This is generally reserved for smaller brain tumors, although stereotactic body radiosurgery has been developed in recent years. SRS is accomplished by delivering multiple small beams of radiation in order to deliver the prescription dose to the target but a much lower dose to the surrounding tissues. Precision is of paramount importance for SRS, and typically rigid frames and fiducial markers are used. Several delivery systems exist for photon-based SRS (e.g., Gamma-Knife, X-knife, Cyber-Knife). Protons can also be used to deliver SRS. Stereotactic treatments may also be delivered over several fractions; this is generally referred to as SRT (stereotactic radiation treatment) . Figure 48-8 shows an SRS plan.
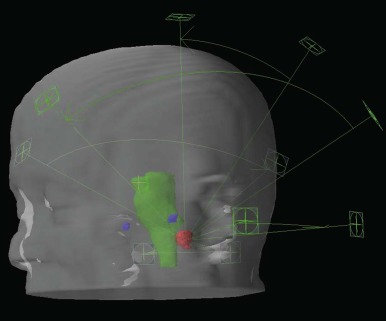
Proton-Beam Radiation
Proton-beam radiation is a form of particle radiotherapy that allows for deposition of maximum dose at a great range of depths (up to 32 cm) and complete sparing of tissues beyond the area of maximum dose deposition. This is in contrast to photons that are limited in the depth at which they deliver maximum dose (less than 3 cm) and continue to deposit the dose until passing through and exiting the body. A single proton beam delivers a small and relatively constant dose until near the end of its range, when the majority of the dose is delivered in the Bragg peak. Beyond this point, no dose is delivered. Because a single Bragg peak covers only 0.5 to 1 cm, multiple Bragg peaks are required to treat a tumor. CT planning is required for proton planning, because the impact of tissue inhomogeneity is greater and the density of the tissue must be taken into account by converting Hounsfield units to proton stopping power. For clinical treatment, proton beams are either combined to form a spread out Bragg peak (SOBP; 3D conformal/scattered proton treatments), or multiple proton beams are scanned across the target volume (scanning beam technique). 3D conformal radiotherapy (CRT) with protons requires patient-specific hardware to shape, or conform, the beam to the target. Lucite compensators shape the distal edge of the beam to the target volume and correct for changes in tissue density, and brass apertures or multileaf collimators shape fields to the target volume laterally. Figure 48-9 shows the delivery of 3D CRT proton treatment for a child using brass apertures and Lucite compensators. When multiple inhomogeneous scanning beam fields are used to treat a target volume, the term intensity-modulated proton therapy (IMPT) is used. Figure 48-10 demonstrates the use of multiple inhomogeneous beams for an IMPT plan. Proton radiation is prescribed in gray radiobiological equivalents (Gy [RBE]) as opposed to Gy, which is used for prescribing photon radiation. The Gy (RBE) takes into account a slightly higher average relative biologic effectiveness. Therefore the prescribed dose and biologic effects of protons are predicted to be equivalent to photons. The difference between protons and photons is the physical dose distribution. These physical properties of protons allow for a reduction in both high and low doses of radiation exposure.
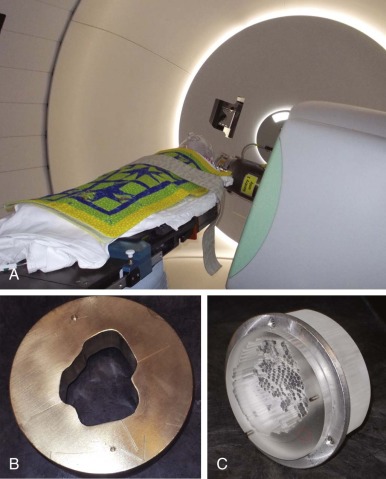
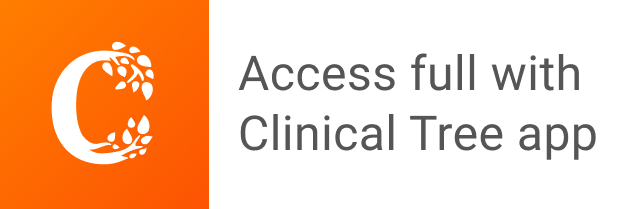