Chapter 10 Nuclear Medicine
Positron Emission Tomography
Basic Physics of Positron Emission Tomography
In addition to delivering anatomic information, the CT portion of PET/CT is used to measure the attenuation of the x-ray photons traveling through the patient to produce the so-called attenuation map and correct the PET data for tissue attenuation. During PET acquisition, photons from structures deep in the abdomen or pelvis are more strongly attenuated than those from superficial structures and the chest. The intensity of uptake in deeper structures is underestimated on non–attenuation-corrected PET images; the intensity of uptake in the deeper structures is normalized to the intensity of uptake in the superficial structures on the attenuation-corrected PET images (Fig. 10-1). Although correction of the PET data for tissue attenuation is indispensable, misalignment between PET and CT can cause mislocalization of lesions on the fused PET/CT images. This may be caused by the changed position of a body part (e.g., neck, legs) or physiologic changes in the position of an organ (e.g., respiratory movement) between the transmission scan (CT) and the emission scan (non–attenuation-corrected PET). Because the degree of misalignment and resulting mislocalization can be significant, the physician must be cautious when interpreting the attenuation-corrected PET/CT images or using PET/CT images for radiation therapy planning. The magnitude of this misalignment can be assessed by fusing the non–attenuation-corrected PET images with the CT images; this can be performed on any PET review station. In case of significant misalignment, the non–attenuation-corrected PET images should be reviewed without fusion with the CT images, and the metabolic findings of PET should be correlated side by side with the anatomic findings of CT (Fig. 10-2).
General Aspects of Tumor Visualization on 18F-Fluorodeoxyglucose Positron Emission Tomography
18F-fluorodeoxyglucose is also taken up in benign processes such as infection and inflammation because white blood cells and fibroblasts are highly avid for FDG. This is probably the most common reason for false-positive findings on PET scans obtained for oncologic indications. A false-positive reading on PET can partly be avoided by obtaining the patient’s medical history and determining the pretest likelihood of an infectious or inflammatory process. There are no published data on the time interval after an invasive procedure or radiation therapy in which a PET scan can be falsely positive, and the inflammatory cell reaction after such a procedure varies among organs. For example, there is evidence that only minimal reactive cell accumulation occurs in the liver within the first 3 days after radiofrequency ablation and that the reactive cells do not cause false-positive PET findings at least in the first 7 days after radiofrequency ablation.1
The standardized uptake value (SUV) is a semiquantitative measure of the tracer uptake in a region of interest that normalizes the lesion activity to the injected dose and body weight; SUV does not have a unit. Despite initial enthusiasm, it is generally accepted that SUV should not be used to differentiate malignant from benign processes, and that the visual interpretation of PET studies by an experienced reader provides the highest accuracy. There are many factors influencing the calculation of SUV, such as the body weight and composition, the time between tracer injection and image acquisition, the spatial resolution of the PET scanner, and the image reconstruction algorithm. Nonetheless, SUV may be useful as a measure to follow the metabolic activity of a tumor over time within the same patient and to compare different subjects within a research study under defined conditions. For example, the SUV of an individual tumor can be measured before and at different time points after therapy, and any change can be used as an index of therapeutic response. However, it is unclear whether this measure can be used for clinical decision making. Some indicate that the intensity of FDG uptake by itself can assess the aggressiveness of a tumor and therefore correlate with prognosis, regardless of the treatment modality. However, there is no firm evidence that patient management should be modified on the basis of intensity of uptake.2
Most Common Indications for 18F-Fluorodeoxyglucose Positron Emission Tomography in Oncology
Lung Cancer
Positron emission tomography has an overall sensitivity of more than 90% and a specificity of about 85% for diagnosing malignancy in primary and metastatic lung lesions; the sensitivity and specificity of PET for small cell lung cancer are similar. The sensitivity of PET for bronchoalveolar lung cancer and carcinoid of the lung is about 60%, and the specificity of PET for lung cancer is lower in areas with a high prevalence of granulomatous lung disease. PET is particularly useful in patients with a low (5% to 20%) or intermediate (20% to 70%) risk of lung cancer, as determined by an evaluation of symptoms, risk factors, and radiographic appearance. In these cases, PET is helpful in moving the patient to the very-low-risk (<5%) or high-risk (>70%) category.3 It is expected that the use of PET for diagnosing malignancy in indeterminate lung nodules will continue to grow as more patients are diagnosed with nodules on CT performed for other indications or as a screening test. Most current PET scanners are capable of detecting lung lesions as small as 6 mm, and the resolution of PET is likely to improve. Most of the literature in this regard is based on data from the 1990s, when the resolution of PET was above 1 cm; studies are being conducted to systematically assess the sensitivity and specificity of PET for detecting malignancy in subcentimeter lung lesions.
In mediastinal staging of non–small cell lung cancer (NSCLC), patients with clinical stage I and II disease have by definition a radiographically negative mediastinum. However, in patients with central tumors, adenocarcinoma, or N1 lymph node enlargement, the false-negative rate of CT for mediastinal involvement is 20% to 25%. It is unclear whether PET should be used instead of mediastinoscopy in staging the disease of these patients. In mediastinal staging of clinical stage III tumors, positive results of PET need to be confirmed by tissue diagnosis because of a relatively high false-positive rate (15% to 20%). The false-negative rate of PET and mediastinoscopy in assessing enlarged mediastinal lymph nodes is 5% to 10%, and some authorities therefore do not pursue biopsy in the case of a negative PET result for disease in the mediastinum, whereas others argue that mediastinoscopy can detect microscopic metastases, and they are not comfortable accepting a negative PET result.4 Practically, in larger centers, patients with stage III tumors undergo both PET to assess for distant metastases and mediastinoscopy, but a strong argument for staging of the mediastinum with PET can be made in communities without an experienced mediastinoscopy service.
For patients with clinical stage I peripheral tumors, most authorities do not request mediastinoscopy before surgery, because the rate of mediastinal or systemic involvement is very low (about 5%); however, this detection rate is based on investigations before implementation of PET, and PET may increase the detection rate. Among patients with clinical stage II tumors, the rate of metastatic disease is higher, and PET may be warranted in these patients to assess for systemic disease. For stage III tumors, the false-negative rate of clinical evaluation for systemic disease is 15% to 30%, and PET is justified instead of a battery of other tests (e.g., bone scan, CT, MRI) to assess for distant metastases.4 PET is more sensitive (90% vs. 80%) and more specific (90% vs. 70%) than bone scan in detecting bone metastases from NSCLC; PET has a sensitivity and specificity of greater than 90% in detecting adrenal metastases from NSCLC. Brain CT or MRI is still needed because PET cannot reliably detect brain metastases because of physiologically intense brain uptake of FDG. For patients with stage IV tumors, PET may be able to indicate the most accessible site for biopsy.
PET is also useful in restaging NSCLC. In particular, PET appears to be more sensitive than CT in differentiating postirradiation change from local recurrence, although differentiating these two entities remains a challenge. The postirradiation change in the chest can remain intense on PET for up to several years. In differentiating local recurrence from postirradiation change, the intensity of uptake and its shape should be taken into account (Fig. 10-3).
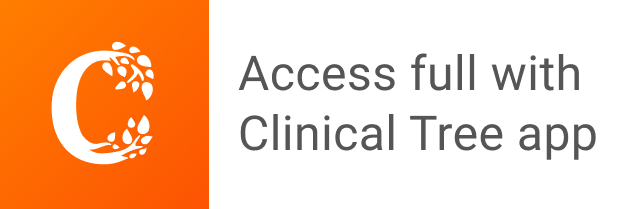