(1)
Université de Bordeaux, Bordeaux, F-33076, France
(2)
CNRS, INCIA, UMR 5287, F-33400 Talence, France
(3)
INSERM U1212, UMR CNRS 5320, Bordeaux, F-33076, France
Keywords
NanoparticlesCarbon-based nanomaterialsMetal-based nanoparticlesNucleolipidsRadionuclide imaging22.1 Introduction
Nanoparticles and nanoscale technologies have gained great interest in biomedical fields, and their use for the treatment, diagnosis, monitoring, and control of biological systems has been referred to as “nanomedicine” by the National Institutes of Health [1]. Nanoparticles mainly employed in nanotechnology usually refer to materials with dimensions less than several hundred nanometers. With the size comparable to biological molecules such as antibodies, enzymes, and receptors, and about 100–10000 times smaller than human cells, these small structures can extravasate through the endothelial cell layers and can be designed to interact with biomolecules both on the surface of or inside the cells. Nowadays nanoparticles can be designed to improve delivery efficacy and in vivo stability of drugs by varying the size, charge, or surface modification. For example, nanoparticles are typically coated with polyethylene glycol to reduce their uptake by the reticuloendothelial system and to increase their plasma half-life. Another noteworthy property of nanoparticles is their very high surface-to-area volume ratio, which enables surface chemistry for targeting but also high loading capacity for diagnostic or therapeutic payload. Hence, several nanoparticles for radionuclide imaging [2–8] and therapy [9–11] featuring different functionalities have been investigated (Fig. 22.1).
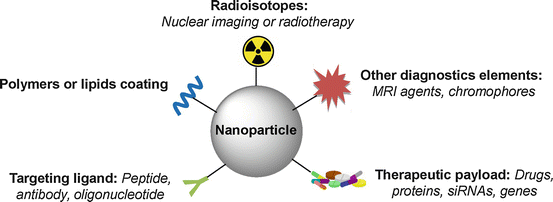
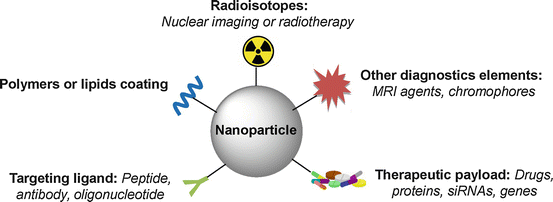
Fig. 22.1
Schematic drawing of nanoparticles used for multimodal radionuclide imaging and therapy
These nanoparticles can be classified according to their chemical composition (Fig. 22.2). The following families can be distinguished: (1) metal-based nanoparticles including quantum dots, iron oxide, and gold nanoparticles, (2) carbon-based nanoparticles such as nanotubes or fullerenes, (3) polymer nanoparticles such as nanocapsules or dendrimers, (4) lipid-based nanoparticles including liposomes and solid lipid nanoparticles, and (5) a new class based on nucleolipid nanoparticles (Fig. 22.2). Two main radiolabeling strategies for nanoparticles have been investigated. One is to radiolabel the nanostructure itself, either on the surface or in the core. The other approach is to radiolabel the payload encapsulated inside the nanoparticles.
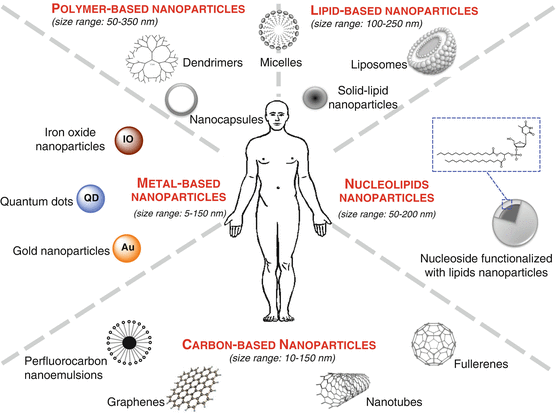
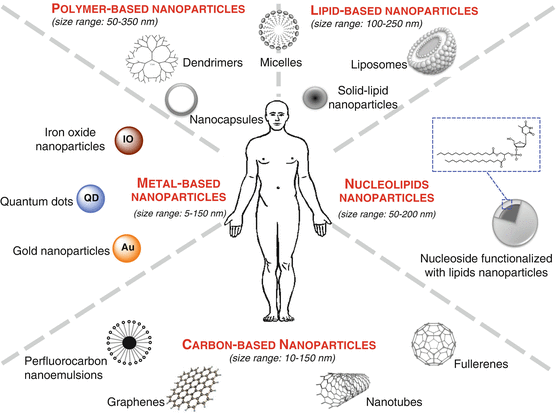
Fig. 22.2
Schematic illustration showing the nanoparticles classified according to their chemical composition
One main concern in the design of nanoparticles for nanomedicine is the efficacy of the targeting to minimize size effect during therapy or false positive in diagnosis. Two approaches are generally used: a passive or an active targeting. In passive targeting, nanoparticles can take advantage of the increased vascular permeability and pass through the leaky vasculature of tumor bed but not transport through tight inter-endothelial junctions in normal tissues. Such spontaneous accumulation is known as the enhanced permeability and retention effect (EPR effect). In active targeting, molecules such as antibodies or specific ligands can be attached to the surface of the nanoparticles to interact with receptors that are overexpressed on tumor or other cells resulting in enhanced accumulation and preferential cellular uptake through receptor-mediated process.
Consequently one major application of nanomedicine is in the field of cancer. Many systems were primarily designed for therapeutic purposes especially for a more efficient delivery of chemotherapeutic drugs to pathologic sites while reducing their accumulation in healthy tissues. Thus, several therapeutic nanoparticles are routinely used in clinic including Doxil (encapsulation of doxorubicin), Ambisome (amphotericin B), or Abraxane (paclitaxel) [12, 13].
The aim of this review is to highlight some of the recent advances in the area of nanoparticles for radionuclide imaging and therapy. We wish to underline that these nanoparticles offer new perspectives in the fields of biomedicine. First, we will focus on the previous works realized on carbon-based nanoparticles. This section includes several examples of fullerenes, carbon nanotubes, graphene oxide, and perfluorocarbon emulsions. Second, we present several metal-based nanoparticles currently developed for nanomedicine. Third, polymer-based nanoparticles are presented with the example of dendrimers. The fourth section is dedicated to the lipid-based particles. The last section underlines recent examples of investigations on promising bioinspired nanoparticles constructed via the supramolecular assemblies of hybrid nucleoside lipids (nucleolipids).
22.2 Carbon-Based Nanoparticles
In recent years, carbon-based nanomaterials have attracted considerable attention in nanomedicine due to their unique properties. These materials such as fullerenes, carbon nanotubes, graphene oxide, and perfluorocarbon nanoemulsions provide unique advantages and opportunities for drug delivery, therapeutics, diagnostics, and imaging.
22.2.1 Fullerenes
The discovery of the buckminsterfullerene (C60) by Kroto et al. in 1985 gave rise to carbon technology era [14]. Fullerene’s structures are composed of closed carbon cages formed by 12 pentagons and a number of hexagons that increased with the number of carbon [15]. Their unique chemical and physical properties make them attractive candidates for diagnostic, therapeutic, and theranostic applications [16, 17]. Several studies report radiolabeling of water-soluble C60 [18–24]. Nikolić et al. developed a novel method for radiolabeling nanoC60 with radioactive iodine, 125I. Biodistribution studies indicated higher accumulation for 125I-nanoC60 compared to the radioactive tracer Na125I in the liver and spleen and also lower accumulation in the thyroid, stomach, lungs, and intestines. The described radiolabeling method might offer opportunities for cancer radiotherapy applications by using 131I instead of 125I [25]. Shultz et al. described the encapsulation of 177Lu, gamma emitter used in SPECT imaging, in a fullerene cage. This agent was conjugated with an interleukin-13 (IL13) peptide to target an overexpressed receptor in gliobastoma multiform tumors. By complexation of other radioisotopes such as 166Ho and 90Y, other diagnostic and therapeutic applications might be elaborated using that kind of nanoplatform [26]. More recently, the same team developed a metallofullerene radiolabeled with lutetium 177, 177Lu-DOTA-f-Gd3N@C80. This theranostic agent is able to deliver effective brachytherapy (with 177Lu) and imaging functionality (MRI exam with Gd). The efficacy of this agent was demonstrated on orthotopic xenograft brain tumor models of glioblastoma multiform (GBM) [27,28]. Alternatively, Diener et al. opted to use 212Bi, an α-emitter, for radio-immunotherapeutic purpose. In order to overcome its short half-life (t 1/2 = 60.6 min), they prepared a C60 fullerene radiolabeled with its parent isotope, 212Pb (t 1/2 = 10.6 h). This nanoplatform was synthetized by recoil following α-decay of its short-lived radioisotope parent 216Po generated in situ by the decay of 224Ra. A preliminary biodistribution study demonstrated that encapsulation of 212Pb in C60 prevented bone accumulation of 212Pb but showed a rather slow clearance. Another limitation highlighted is that 36 % of the 212Bi formed was released from the fullerene during the β-decay of 212Pb [29].
22.2.2 Carbon Nanotubes
Carbon nanotubes have been first described by Ijima in 1991 [30]. They are homogenous tubes of rolled graphene sheets between 50 nm and 1 cm diameter. Nanotubes are classified in two different categories according to the number of graphene layers: (i) the single-walled carbon nanotube (SWCNT) from 1 to 2 nm diameter and (ii) the multi-walled carbon nanotube (MWCNT) from 10 to 100 nm diameter [31]. Different carbon nanotubes are used in imaging or therapy [32]. Mc Devitt et al. functionalized an SWCNT with a DOTA derivative for the introduction of 86Y and 111In, radioisotopes used in PET and SPECT, respectively. Whole-body PET images showed an accumulation of radiotracer essentially in the liver, intestine, spleen, and at a lower level in bones [33]. Thereafter, a similar SWCNT was synthetized by the same team with a DOTA macrocycle grafted for 111In chelation and also an antibody targeting CD20 epitopes overexpressed in Burkitt’s lymphoma human cells. This resulting tracer succeeded in selectively targeting in vitro tumoral cells and in vivo tumors [34]. Villa et al. synthesized two new SWCNTs with two moieties (i) a single-stranded oligonucleotide, which undergoes self-assembly in the presence of the complementary-stranded, and (ii) a radiotracer component, made up of a 111In-DOTA and a targeting component, consisting of an RGD peptide [35]. Liu et al. developed an SWCNT functionalized with PEG phospholipids. In this study, they attached DOTA macrocycles for 64Cu introduction and c(RGDyK) peptides in order to target positive αVβ3 integrin tumors (U87MG) in mice. The high tumor uptake of the 64Cu-SWCNT-PEG-RGD visualized by PET imaging was attributed to the multivalent effect (several RGD grafted by nanotubes). Raman spectroscopy revealed the presence of SWCNT-PEG-RGD in the liver and tumor and at a lower level in the kidney, but no SWCNT Raman signal was observed in the muscle [36]. Ruggiero et al. developed neo-vessels and irregular tumor vessels targeting nano-objects. For this purpose, this team synthetized two new functionalized SWCNTs with endothelial angiogenic cell-specific antibody, E4G10. The first derivative was composed of a DFO chelate allowing 89Zr chelation to follow its accumulation by PET imaging. The second derivative was a DOTA chelate for 225Ac introduction, α emitters used in radiotherapy. This study showed that those two nano-objects were specific to the targeted vessels. SWCNT-DFO89Zr-E4G10 gave PET imaging with a good contrast, and SWCNT-DOTA225Ac-E4G10 presented a significant therapeutic effect [37].
22.2.3 Graphene Oxide
Graphene oxide (GO) is made of monoatomistic carbon atom layers in a honeycomb structure. Graphene oxide has excellent electrical, thermal, mechanical, and optical properties [38]. In addition to their hydrophilic properties, their surface can be easily functionalized with, for example, hydrophilic polymers. GO has carefully been studied for biomedical applications in numerous studies [38–41] more particularly for imaging and therapeutic purposes [42–45]. Shi et al. synthetized a reduced graphene oxide (RGO) conjugated to two moieties: (i) an antibody TRC105 anti-CD105 and (ii) a 64Cu-labeled NOTA macrocycle, allowing tumor vasculature targeting and PET imaging on mice model of breast cancer. 64Cu-NOTA-RGO-TRC105 showed excellent in vitro and in vivo stability and vascularized tumor specificities [46]. More recently, this team used a new 64Cu-labeled GO derivative, functionalized with a VEGF ligand to enhance tumor vasculature targeting. In vivo studies were done on mice with U87MG tumors. 64Cu-GO-VEGF nanoconjugate showed effective targeting for VEGFR on U84MG tumor models and showed great stability [47]. Chen et al. described the design and synthesis of a PEG-coated reduced nano-graphene oxide (RGO) labeled with 131I. This RGO derivative had the advantage of giving access to radiotherapy and photothermal therapy simultaneously. After a 10 mg/kg 131I-RGO-PEG (200 uCi) dose-injection on mouse tumor models, an effective elimination of the tumor after irradiation at 800 nm for 20 min had been shown. Toxicological data revealed no toxicity of the 131I-RGO-PEG derivative at administrated dose on treated mice [48]. Another interesting idea developed by Fazaeli et al. was to radiolabel GO-aminopropylsilyl derivative with 198/199Au nanoparticles to target and visualize by SPECT imaging fibrosarcoma tumors. This nanoconstruct allowed a fast and effective tumor visualization. However, the low lipophilicity of this structure induced also a fast body excretion (24 h) through kidneys [49].
22.2.4 Perfluorocarbon Nanoemulsion
Perfluorocarbon nanoemulsions are composed of perfluorocarbon liquid encapsulated in a phospholipidic monolayer and dispersed in water. Those particles are widely used for medical applications, more precisely for drug delivery [50,51], atherosclerosis [52], tumors, and plaque angiogenesis [53] diagnostic and treatment. For imaging, perfluorocarbon derivatives are widely studied as 19F MRI contrast agents [54–56] as well as bimodal 19F MRI-optical imaging agents [57–60]. Few studies also reported an application for PET imaging. In this context, Hu et al. developed 111In-labeled perfluorocarbon nanoparticles for αVβ3 integrin targeting and cancer-induced angiogenesis detection. The in vivo studies were done on Vx-2 rabbit tumors. Nanoparticles provided a high tumor signal and accumulation in nascent tumors as a function of both specific and passive entrapment within the neovasculature [61]. More recently, Fabiilli et al. encapsulated 18F-fluorodeoxyglucose (FDG) in a double perfluorocarbon emulsion and performed emulsion or solution injections in rats bearing gliosarcoma. When FDG solution was compared to FDG emulsions, FDG uptake decreased by 36 % in the brain and 44 % in tumors (calculated from the AUCSUV). Moreover, dynamic micro-PET imaging indicated no accumulation of FDG emulsions in the lungs, which is critical in minimizing PFC-related bioeffects [62].
As we briefly resumed in this section, carbon-based nanomaterials possess intrinsic physicochemical properties that are wisely exploited. However, potential toxicity of those nanomaterials has been pointed out in the last decade and is well highlighted and described in extensive toxicological review and studies [63–68]. Size, shapes, surface properties and chemistry, concentration, agglomeration, dose, and preparation of carbon-based nanomaterials are all determinants for their biological activities that may also lead to toxicity.
22.3 Metal-Based Nanoparticles
22.3.1 Gold Nanoparticles
In biomedical field, gold nanoparticles have received increasing attention due to their biocompatibility and optical properties, conferred by their localized surface plasmon resonance (SPR) in particular in imaging (x-ray [69] and photoacoustic [70] imaging) and cancer therapy [71–73]. Gold nanoparticles (AuNPs) consist of a Au core with surface coating. They are colloidal or clustered particles with diameters in the range of a few to several hundred nanometers. In order to control optical properties, size and shape can be easily tuned to obtain 1 nm to 150 nm AuNPs with diverse morphologies. Recent studies have reported AuNPs radiolabeling for therapy [74, 75] and nuclear imaging [76–86].
Karmani et al. studied conjugation influence of the distribution of an antibody, the cetuximab on gold nanoparticle labeled with 89Zr in mice bearing A431 xenografts. Immuno-PET studies showed that conjugation of cetuximab to AuNP did not affect EGFR-dependent tumor uptake. However, compared to 89Zr-cetuximab, the higher reticuloendothelial system uptake for AuNP-89Zr-cetuximab could be a limitation of radioactive nanoparticle use for systemic metabolic radiotherapy [79].
Felber et al. described a new coating ligand for Qds and AuNPs allowing direct labeling with [99mTc(OH2)3(CO)3]+ in the last step. The ligand comprises (i) a thiol group as anchor for the NPs surface, (ii) a PEG linker, (iii) and 2,3-diaminopropionic acid to act as chelator for [99mTc (CO)3]+ and for the introduction of a small prostate-specific membrane antigen (PSMA) inhibitor via an amide link. Biodistribution of various sized (7 and 14 nm) AuNPs performed by micro-SPECT showed rapid clearance from the blood pool through hepatobiliary pathway, in mice bearing LNCaP xenografts. 7 nm AuNPs revealed higher stability and significantly higher bone uptake than 14 nm AuNPs [82].
Zhang et al. reported functionalized gold nanoparticles preparation through nucleic acid-directed assembly. Oligodeoxynucleotide-derivative gold nanoparticles were assembled with a library of pre-functionalized complementary PNAs (peptide nucleic acid). PNAs were functionalized with either (i) 64Cu-DOTA for PET imaging, (ii) PEG for stealth properties, or (iii) with Cy5 for fluorescent imaging. The resulting AuNPs showed a good in vitro and in vivo stability [84]. Another interesting strategy developed by Black et al. consisted in the preparation of multispectral SPECT agent to quantify MMP9 expression in tumors. AuNPs were radiolabeled by two distinct nuclides, 125I and 111In, separated by an MMP9-cleavable peptide [85].
177Lu-labeled AuNPs conjugated to different peptides were proposed as a new class of theranostic agents and have been recently reviewed [74]. In this context, Luna-Gutiérrez et al. evaluated the potential of 177Lu-labeled gold nanoparticle (20 nm) conjugated to cyclo-[RGDfK(C)] peptide via the cysteine thiol group, as a plasmonic photothermal therapy and targeted radiotherapy system in MCF7 breast cancer cells. After laser irradiation, [RGDfK(C)]-AuNPs’ presence in cells caused a significant decrease in MCF7 viability, down to 9 %, and after treatment, with 177Lu-[RGDfK(C)]-AuNP, the MCF7 proliferation was significantly inhibited [87]. They also evaluated 177Lu-[RGDfK(C)]-AuNP therapeutic response in athymic mice bearing αVβ3-integrin-positive C6 gliomas. The nanoconstruct significantly decreased glioma tumor progression. No uptake in nontarget organs or renal toxicity induction was observed [88].
22.3.2 Iron Oxide Nanoparticles
Iron oxide nanoparticles are superparamagnetic particles especially used as MRI contrast agents. Several studies reported their radiolabeling for use in MRI/PET or MRI/SPECT bimodal imaging and have been recently reviewed [89].
22.3.3 Other Metal-Based Nanoparticles
A particularly interesting idea, developed by Pérez-Campaña et al., consists in 18F radiolabeling of 18O-enriched aluminum oxide NPs by direct irradiation with 16 MeV protons. Size and crystalline phase of those NPs were not altered during the irradiation step. Biodistribution studies were conducted in male rats after intravenous injection. PET imaging has shown NP rapid accumulation in the liver. Radioactivity was also detected in the lungs, kidney, heart, and stomach. This labeling strategy is promising for activation of metal oxide particles or powder [90]. A more recent study, published by the same group, presented an analogous strategy for 13N radiolabeling of commercially available aluminum oxide with different sizes via the 16O (p,α)13N nuclear reaction. PET imaging performed after intravenous injection in rats allowed to determine NP accumulation in organs up to 68 min postinjection, despite the short half-life of radioactive 13N. Results have shown that large NPs were uptaken in the lungs, while small particles were accumulated in the stomach, heart, kidney, and bladder. Moreover, a high accumulation in the liver was observed regardless of the NP size (Fig. 22.3) [91].
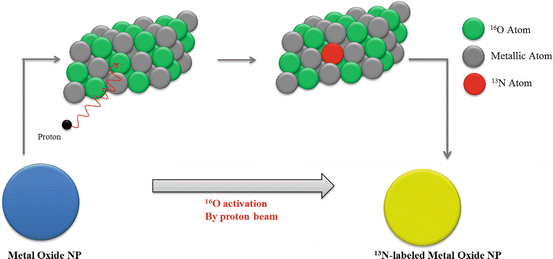
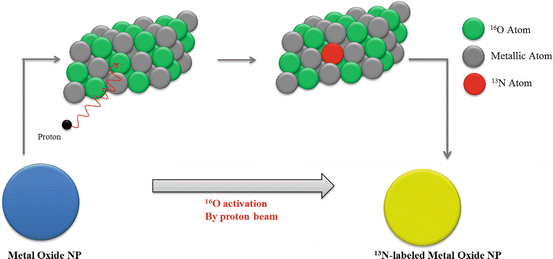
Fig. 22.3
Activation of Al2O3 NPs by proton irradiation via the 16O (p,α)13N nuclear reaction. Metal oxide NPs are directly irradiated with protons, converting 16O atoms to 13N atoms by collision. Adapted from [91]
22.4 Polymer-Based Nanoparticles
22.4.1 Dendrimers
Dendrimers are highly branched macromolecules with a well-defined number of peripheral groups discovered by Tomalia et al. in the early 1980s [92]. They formed spherical object in the nanometer range. Size, architecture, density, and surface groups are easily tunable. They are widely used in nuclear imaging and therapy fields, especially due to the possibility to incorporate several recognition units in order to improve targeting [93]. Moreover in imaging field, dendritic structures are also used to design multivalent or multimodal contrast agents [94]. However, the question of their biodegradability is highly discussed, and biocompatible dendrimer designs are reviewed by Cheng et al. [95].
A PAMAM dendrimer DOTA conjugate was designed by Ghai et al. 68Ga radiolabeling was achieved with high radiochemical yield (79 % decay corrected), and the subsequent tracer presented good stability. Preliminary PET imaging studies in mice bearing EAT (Ehrlich’s ascites tumors) showed a 68Ga-dendrimer accumulation in tumor through passive targeting and excretion through kidney [96].
McNelles et al. reported the synthesis of a PEGylated fifth-generation aliphatic dendrimers functionalized with peripheral vinyl groups and a dipicolylamine Tc(I) chelate core. The radiolabeling was achieved with high radiochemical purity (>99 %) using [99mTc (CO)3(H2O)3]+. SPECT imaging of healthy rats indicated that the radiolabeled dendrimer circulated in the blood up to 24 h postinjection mainly due to PEG chains. SPECT imaging experiments were then performed on xenografted murine tumor model. Results demonstrated a dendrimer accumulation within the tumor through the EPR effect 6 h postinjection [97].
Laznickova et al. described the synthesis of 177Lu-radiolabeled G1 and G4 PAMAM dendrimer functionalized with DOTA-monopyridine-N-oxide. Biodistribution studies on rats revealed that 177Lu-G1-PAMAM dendrimer injection induced a lower residence time of radioactivity in the blood and organs, in comparison with 177Lu-G4-PAMAM dendrimer. However, the latter allowed the conjugation of higher number of metal chelator and thus radiometal per molecule [98]. A recent study achieved by Kovacs et al. described also the synthesis of 177Lu-G4-PAMAM dendrimers functionalized with DOTA to determine the elementary changes in tumors caused by ionizing radiations. C57BL/6 mice tumor tissues treated with 177Lu-DOTA-dendrimer showed decreases in Br, Ca, Cl, K, and Na concentrations and increases in Fe, Mg, Rb, S, and Zn concentrations, when compared to untreated tumors tissues [99].
Zhao et al. developed a 131I radiolabeled theranostic platform for SPECT imaging and radiotherapy of an MMP-2 overexpressing xenografted glioma model in vivo. Generation five amine terminated poly(amidoamine) was used to conjugate (i) PEG, (ii) targeting agent chlorotoxin (CTX), and (iii) 3-(4’-hydroxyphenyl)propionic acid-OSu (HPAO). The HPAO phenol groups allowed 131I radiolabeling with good stability and high radiochemical purity. The 131I-dendrimer biodistribution was studied by SPECT imaging. Dendrimers were highly uptaken in the liver, and a low accumulation was observed in the tumor, lungs, stomach, heart, kidney, spleen, intestines, and soft tissues. It should be noted that tumor uptake was higher for the 131I-CTX-dendrimer in comparison with the 131I-dendrimer without CTX. Those data confirmed the targeting role mediated by the CTX moiety. It was also demonstrated that after treatment with the 131I-CTX-dendrimer, the tumor grows slower than those of the mice treated by either saline, Na131I, or 131I-dendrimer without CTX [100].
22.4.2 Nanocapsules
Nanocapsules are vesicular colloidal system constituted with a liquid core and polymeric shell. Such structures can be obtained from a large variety of monomers or polymers through direct chemical reaction or through precipitation of preformed template at the interface of the droplet. Size, morphology, and functionalization can be controlled [101]. Few examples of radiolabeled nanocapsules with 99mTc have been reported [102–104]. In this context, Pereira et al. radiolabeled nanocapsule with 99mTc-HMPAO (D,L-hexamethylpropyleneamine oxime) in order to identify inflammatory process in rats. Nanocapsules were prepared with biodegradable polymers poly(D,L-lactic acid) (PLA) and were PEGylated or not. The authors demonstrated that both types of nanocapsules were able to encapsule 99mTc-HMPAO. However, as shown by AFM, physical and structural properties of PEGylated nanocapsules were more homogeneous. Biodistribution studies have shown similar uptake in inflamed and control paws for 99mTc-HMPAO-NC and free 99mTc-HMPAO, while PEGylated 99mTc-HMPAO-NC have shown a greater uptake. The authors have concluded that the latter could be useful as radiotracer for inflammatory process imaging [105, 106]. Polyglucosamine-/squalene-based nanocapsules (PG-NC) were radiolabeled with 111In by Vicente et al. to monitor the biodistribution of PG-NC and their affinity for the draining lymph nodes. After injection in New Zealand white rabbits, γ-scintigraphy imaging revealed slower clearance than for the control 111InCl3 and progressive accumulation in popliteal lymph node [107].
22.5 Lipid-Based Nanoparticles
22.5.1 Liposomes
Liposomes are defined as phospholipid vesicles consisting of one or more concentric lipid bilayers with an aqueous internal cavity. With a wide range of physicochemical and biophysical properties (particles size, charge, number of lamellae, lipid composition, surface modification), liposomal formulations can be modified to control their biological behaviors. Liposomal systems have received widespread attention as nanocarriers for a wide range of biomedical applications by overcoming obstacles to cellular and tissue uptake, stabilizing therapeutic compounds, and improving biodistribution of lipophilic and hydrophilic compounds to target sites [108]. Numerous studies described the radiolabeling of liposomes for diagnostic or therapeutic applications [9, 109–111].
Recently De Barros et al. prepared long circulating and pH-sensitive liposomes (SpHL) containing bombesin radiolabeled with 99mTc (99mTc-BBN(7–14)) to target breast cancer tissues. Liposomes presented a diameter in the range of 160 nm and a 99mTc-BBN(7–14) encapsulation percentage around 30 % in the presence of glucose (SpHLG). Moreover the authors demonstrated that the liposomes remained stable up to 120 days of storage. When injected in breast tumor-bearing nude mice, SpHLG-99mTc-BBN(7–14) exhibited a high tumor/muscle and tumor/blood ratio. Furthermore, scintigraphic studies revealed a strong signal in tumor area suggesting the tumor specificity of the radiotracer [112]. Ogawa et al. reported the synthesis of 111In-radiolabeled liposome modified with phosphatidylserine for atherosclerotic plaque imaging. It was demonstrated that the tracer allowed macrophages and artherosclerotic region targeting in Apo E −/− mice. However, too rapid blood clearance was also observed [113]. In order to solve this problem, the authors developed new liposomes-PS modified with PEG. As expected, slower blood clearance was observed with PEGylated liposomes. Nevertheless, PEGylation did not improve the uptake in artherosclerotic plaques in vivo [114]. Bandekar et al. evaluated the potential of PEGylated liposomes radiolabeled with 225Ac to selectively kill PSMA-expressing human (LNCaP and HUVEC induced to express PSMA) and rat (Mat-Lu) cell lines in vitro. Two targeting ligands were compared: (i) the mouse antihuman PSMA J591 antibody and (ii) the A10 PSMA aptamer. J591-liposomes showed higher level of specific binding to all cell lines than A10-liposomes did. It has also been shown that radiolabeled J591-labeled liposomes are more cytotoxic than radiolabeled A10-liposomes. Moreover relatively low LD50 values of J591-labeled liposomes both on LNCaP and on PSMA-expressing HUVEC suggested the potential of these liposomes for selective antivascular α-radiotherapy [115]. Petersen et al. described theranostic liposomes and evaluated their potential in human xenografted mouse model. For this purpose PEGylated liposomes were radiolabeled either with 64Cu for PET imaging or with 177Lu for internal tumor radiotherapy. They investigated also the optimal level of PEGylation in liposomes. The authors demonstrated that 10 % mol PEGylated liposomes showed higher tumor uptake than 5 % mol PEGylated liposomes. PET imaging with 64Cu-liposomes allowed clear tumor visualization. High-absorbed doses to the tumor were estimated by dosimetric analysis for 177Lu-liposomes. This result suggested that 177Lu-liposomes could achieve adequate delivery of therapeutic internal radiation to solid tumors. However, the therapeutic potential of 177Lu-liposomes must be further evaluated in a tumor-bearing mice model [116].
22.5.2 Solid Lipid Nanoparticles
Solid lipid nanoparticles (SLNs) consist in spherical solid lipid particles dispersed in water or in aqueous surfactant solution. They are usually constituted with a hydrophobic solid core coated with a phospholipid monolayer. They are highly used as drug carrier [117–119]. Radiolabeled SLNs have been designed as potential nuclear imaging agent particularly as SPECT imaging agents by 99mTc radiolabeling [120–124]. In a recent study, Banerjee et al. described the synthesis of SLNs encapsulating paclitaxel radiolabeled with 99mTc by a novel method. Taxol was radiolabeled also, in order to compare their biodistribution in normal rats. It was found that brain uptake of 99mTc-SLNs was 5 times higher than 99mTc-Taxol. This result suggests that 99mTc-SLNs are able to deliver more paclitaxel in the brain than Taxol formulation [125]. Andreozzi et al. developed a method for SLNs radiolabeling by 64Cu for PET imaging. A BAT chelator (6-[p-(bromoacetamido) benzyl]-1,4,8,11-tetraazacyclotetradecane-N,N′,N′′,N′′′-tetraacetic acid) was conjugated with a synthetic lipid and incorporated into the SLN monolayer. 64Cu-SLNs were obtained with 67 % radiolabeling yield after incubation with 64CuCl2. PET imaging and gamma counting suggested that 64Cu-SLNs are circulating in the bloodstream after 3 h but are almost entirely cleared after 48 h. The authors planned to coat SLNs with hydrophilic polymers such as PEG to reduce SLNs clearance from the RES and increase the blood half-life [126].
22.5.3 Micelles
Micelles are colloidal dispersions belonging to a group of association or amphiphilic colloids, which form spontaneously under certain concentration and temperature phase. Polymeric and non-polymeric micellar systems are subject of many publications addressing various biomedical applications due to favorable biodistribution and lower toxicity of encapsulated therapeutic agents [127]. Radiolabeled micelles have been extensively studied during the last few years [128–140]. A recent study described the use of lipid-coated magnetite micelles to act simultaneously as vehicle for vaccine and as radiotracer for SPECT/PET imaging. Similarity between Ga3+ and Fe3+ in charge and size allowed achieving gallium radiolabeling by exchange in biomolecule metalation. Antigen (OVA) and TLR9 agonists (CpGS) were accommodated in the hybrid micelles for efficient delivery in the lymph nodes. SPECT imaging demonstrated migration from the injection site to regional and non-regional lymph nodes. In addition, in vitro and in vivo studies showed that cellular and hormonal immune responses were greatly enhanced and provided protection against tumor [141]. You et al. used a combination of core cross-linked polymeric micelles radiolabeled with 177Lu (CCPM-177Lu) and cyclopamine solubilized in lipid-liquid nanoparticle system (CPA-LLP). Cyclopamine is a potent inhibitor of Hedgehog pathway studied for anticancer effect. It has also been investigated for enhancing tumor response to radiation therapy. As expected clonogenic assays demonstrated that CPA-LLP significantly enhances cell response to CCPM-177Lu radiotherapy. Moreover, combination of CPA-LLP and CCPM-177Lu delayed tumor growth more than monotherapy alone, in 4T1 Miapaca-2 mouse xenografted models [142]. Multifunctional theranostic unimolecular micelles have been generated by Guo et al. They conjugated brush-shaped amphiphilic block copolymers to a monoclonal antibody anti-CD105 (TRC105) and to NOTA macrocycle for 64Cu introduction. In addition doxorubicin was loaded into the unimolecular micelle. It has been demonstrated that these multifunctional unimolecular micelles offer pH-responsive drug release and noninvasive PET imaging capability, together with both passive and active tumor-targeting [143].
22.6 Nucleolipid-Based Nanoparticles
The self-assembly properties of biomolecules play a fundamental role in biology, and these principles have been widely exploited for the implementation of supramolecular structures and nanodevices [144]. The multiple cooperative non-covalent interactions (e.g., H-bonding, π − π stacking, hydrophobic effects, etc.) between biomolecules allow the synthesis of complex biological systems and structures. Scientists are taking advantage of these biological principles for the design of hybrid amphiphiles based on the combination of biological units such as amino acids [145]. Peptides [146–149], sugar [150–152], nucleic acids [153, 154], and lipids are currently emerging as new class of biocompatible molecules for biomedical applications. The combination of lipid with nucleoside/nucleotide moieties has been reported to improve the cellular internalization of nucleoside drugs [155] in the treatment of certain diseases including cancers. Nucleolipids [156–158] have been the object of intense investigations due to their unique self-organization properties and their potential applications in the biomedical field. For example, nucleolipids featuring reduced cytotoxicity have been used for the transfection of DNA [159] and siRNA [160, 161].
Ten years ago our group has reported the formation of microspheres simply by hydrating phosphocholine-based nucleolipids with aqueous solutions containing either actinide or lanthanide salt [162, 163]. These actinide and/or lanthanide microspheres were prepared as independent objects suspended in aqueous media. Such a result was the first step in the exploration of nucleolipid behaviors in the presence of radionuclides. The formation of these microspheres demonstrated the versatility and utility of nucleolipids in this field and the importance of combining nucleolipids and inorganic metals to create new structures for the synthesis of nano- and microscale materials using the principles of supramolecular assembly.
99mTc has long track records for use in drug development as SPECT imaging agents [164]. Considering the above and the potential of 99mTc for labeling nanoparticles, recently, we investigated advanced formulations featuring nucleolipids. Interestingly, it was demonstrated that nucleolipid-based nanoparticles can be labeled with 99mTc in the absence of chelating agent such as DOTA (1,4,7,10-tetraazacyclododecane-1,4,7,10-tetraacetic acid). These nanoparticles were implemented using a bottom-up strategy involving positive, negative nucleoside lipids (NLs) and PEGylated nucleoside lipids (Fig. 22.4). The direct labeling of these nanoparticles was realized with 99mTc using stannous chloride as a reducing agent to reduce pertechnetate oxidation state from +7 to +5. We hypothesized that in these conditions 99mTc forms a complex with uridine moieties (Fig. 22.5). The labeling efficiency was found to be quantitative (yield higher than 97 %). Importantly, it was shown that such functionalized and labeled nanoparticles loaded with cisplatin (active principle ingredient, API) can address pharmacokinetic and biodistribution issues. Indeed, it was found that these nanoparticles increase the half-life of the API in vivo and accumulate in tumor tissue, indicating that nucleolipid-based formulations could provide novel radiotracer labeled nanoparticles with potential applications in the field image-guided therapies.
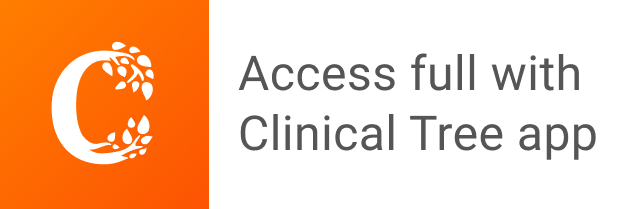