Abstract
Fine-needle aspiration cytology (FNAC) represents the gold standard for the evaluation of thyroid nodules, although 20% to 30% of FNAC have indeterminate cytology, recognizing the problem of the correct management of these patients. Molecular testing on FNAC for at least BRAF, RAS, PAX8-PPRγ, and RET/PTC helps in refining diagnosis, thus contributing to avoiding unnecessary thyroidectomy.
Keywords
oncogene mutations, rearrangements, diagnosis, thyroid nodules, indeterminate lesions
Introduction
Thanks to great technological advances, our understanding of the genomics of thyroid cancer has dramatically improved over the last few years. A number of mutations have been demonstrated in differentiated thyroid cancers, and some of them are considered driver mutations for specific cancer histotypes, often configuring genotype-phenotype relationships.
Genetic events include somatic missense mutations or rearrangements of oncogenes in the MAPK and phosphatidylinositol-3 kinase (PI3K)/AKT signaling pathways.
Oncogene rearrangements
The most frequent oncogene rearrangement is RET/PTC. More rarely, TRK rearrangements, ALK rearrangements, and PAX8/PPARγ rearrangements are found.
RET/PTC
The Ret proto-oncogene is located at chromosome 10 (10q11.2) and encodes a tyrosine kinase cell surface receptor for members of the glial cell line-derived neurotrophic factor family. All these factors activate RET via different glycosyl phosphatidylinositol-linked GFRα receptors. Ret gene alternative splicing results in the production of three different isoforms named RET51, RET43, and RET9, which contain 51, 43, and 9 amino acids in their C-terminal tail, respectively. The RET51 and RET9 are the most common isoforms in which RET is found in vivo. Ret protein is composed of an N-terminal extracellular domain with four cadherin-like repeats, a cysteine-rich region, nine N-glycosilation sites, a hydrophobic transmembrane domain, and a cytoplasmic tyrosine kinase domain.
Ret activates the Raf/Mek/ERK1/2 cascade, which regulates cell proliferation, and the PI3K/Akt signal transduction pathway, which regulates cellular survival. Normally Ret is expressed at very low levels in thyroid follicular cells, but the chimeric forms of Ret are constitutively active due to the fusion of the C-terminal kinase domain of Ret with the promoter and N-terminal domains of unrelated genes. Several rearrangements have been described: inversion inv (q11.2;q21) with H4 gene (D10S170 locus) generates the RET/CCDC6 (PTC1) oncogene; inversion inv (q11.2;q11.2) with ELE1 gene generates the RET/NCOA4 (PTC3) oncogene; translocation t(10;14)(q11;q32) with GOLGA5 generates the RET/GOLGA5 (PTC5) oncogene; translocation t(8;10)(p21.3;q11.2) with PCM1 generates the PCM1/RET fusion; translocation t(6;10)(p21.3;q11.2) with RFP generates the Delta RFP/RET oncogene; translocation t(1;10)(p13;q11) with TRIM33 generates the TRIM33/RET (PTC7) oncogene; and translocation t(7;10)(q32;q11) with TRIM24/TIF1 generates the TRIM24/RET (PTC6) oncogene. The most common rearrangements are RET/PTC1 and RET/PTC3, accounting for 10–20% of all papillary thyroid carcinomas.
TRK
Neurotrophic tyrosine kinase receptor type 1 (NTRK1) gene, also named TRK, is located on the q arm of chromosome 1 (1q21-22) and encodes for a member of the neurotrophic tyrosine kinase receptor (NTKR) family. This kinase is a membrane-bound receptor that, upon neurotrophin binding, phosphorylates itself and members of the MAPK pathway leading to cell differentiation. Genes involved in TRK rearrangements are at least three: TPM3 located at 1q22-23, TGF located at 3q11-12, and TPR located at 1q25. Namely, TRK is a rearranged protein between NTRK1 and TPM3; TRK1 and TRK2 are chimeric proteins between NTRK1 and TPR; and TRK3 is the rearranged product of NTKR1 and TGF. TRK rearrangements are found in 1–5% of papillary thyroid carcinomas.
ALK
The ALK rearrangement results from the fusion of the striatin, calmodulin-binding protein (STRN, 2p22.2) gene and the anaplastic lymphoma kinase (ALK, 2p23.1) gene. The first one functions as a signaling protein and appears to be involved in dendrite development, tight junction assembly, and regulation of cell proliferation. The ALK gene is implicated in phosphorylation of several substrates, including the MAP kinases MAPK1/ERK2 and MAPK3/ERK1. ALK also acts as a receptor for the ligands pleiotrophin (PTN), a secreted growth factor, and midkine (MDK), a PTN-related factor, thus participating in PTN and MDK signal transduction.
The ALK rearrangement is present in about 9% of poorly differentiated thyroid cancers, 4% of anaplastic thyroid cancers, and 1% of papillary thyroid cancers.
PAX8/PPARγ
The PAX8/PPARγ rearrangement consists in a fusion of the coding region of the paired box 8 (Pax-8) and of the coding region of the PPARγ (peroxisome proliferator-activated receptor gamma). The PAX8 gene is located on chromosome 2 and is a member of the paired box (PAX) family of transcription factors. The gene encodes for a nuclear protein, which contains a paired box domain, an octapeptide, and a paired-type homeodomain. Five different isoforms of the protein have been described due to alternative splicing: isoform a (450 a.a.); isoform b (387 a.a., lack exon 8); isoform c (398 a.a., lack exons 7, 8); isoform d (321 a.a., lack exon 8); and isoform e (287 a.a., lack exons 8, 9, 10). Pax8 is expressed in embryonal human tissues, in particular in the developing thyroid gland and kidney and only in these tissues does it remain expressed in adults. The PPARγ gene is located on chromosome 3 at position p25. The encoded proteins are a group of nuclear receptor proteins that function as transcription factors regulating expression of genes involved in cellular differentiation, development, and metabolism. The PAX8/PPARγ rearrangement is found in 30–40% of follicular carcinomas and at lower frequency in the follicular variant of papillary thyroid carcinoma. The molecular event found in thyroid cancer is represented by an interchromosomal translocation t(2;3)(q13;p25), which fuses promoter elements of the PAX8 gene with most of the coding sequence of the PPARγ gene. PAX8 can break at the junction between exon 7 and exon 8, exon 8 and exon 9, or exon 7 and 9 (losing exon 8) and fuse with exon 1 of the PPARγ gene.
Gene mutations
Somatic missense point mutations involve mainly the RAS gene family (H-, K-, and N-RAS), the BRAF gene, and the human telomerase reverse transcriptase (hTERT) promoter.
RAS Isoforms
RAS genes (H-RAS, N-RAS, and K-RAS) encode for four different proteins (one H-Ras, one N-Ras, and two K-Ras) approximately of 21 kDa, which are involved in cellular differentiation, division, and cell death. All proteins have at their C-termini covalently attached lipid tails, composed of farnesyl, palmitol, or geranylgeranyl groups, which enable the Ras proteins to become anchored to the cytoplasmic membrane. When Ras is inactive, it binds a GDP molecule. The Ras signaling cycle starts upon an upstream stimulatory signal. Ras moves from inactive state to an active GTP-binding condition due to guanine nucleotide exchange factor (GEF) stimulation. Then, using its own intrinsic GTPase function, Ras cleaves GTP coming back to its quiescent configuration. Point mutations of Ras block this cycle by inactivating the intrinsic GTPase activity of Ras and blocking the protein in its active state. Studies carried out on the amino acidic sequence of Ras showed that the 12th, 13th, and 61st residues are those mainly affected by point mutations. These amino acidic residues are located around the catalytic GTPase domain of Ras. Consequently, almost all substitutions of these amino acids compromise GTPase function. Activated Ras acts, at the same time, downstream through the PI3K pathway, Raf-MEK-ERK1/2 pathway, and Ral-GEF pathway inducing many of the phenotypic changes involved in neoplastic transformation. RAS mutations are probably an early event in thyroid tumorigenesis and are not tumor specific. They can be found in follicular carcinomas, in the follicular variant of papillary thyroid carcinomas, in poorly differentiated thyroid cancers, but also in follicular adenomas.
BRAF Oncogene
The BRAF (v-raf murine sarcoma viral oncogene homolog B1) gene is located on chromosome 7 and encodes for an 18-exon cytoplasmic protein, which is recruited to the membrane upon growth factor stimulation. It is a member of the Raf kinase family of serine/threonine-specific protein kinases. BRAF interacts with other proteins such as HRAS, AKT, CRAF, and it has been demonstrated to play a role in cell growth, differentiation, and survival through ERK1/2 activation. More than 30 mutations of the BRAF gene associated with human cancers have been identified, and these mutations are mostly located within the part of the gene encoding the kinase domain, clustered to two regions containing the glycine-rich P-loop of the N-lobe and the activation segment. Among these mutations, the V600E (T1799A), resulting in a T-to-A transversion at position 1799 with a valine-to-glutamate substitution, is the most common BRAF mutation in papillary thyroid cancers (about 45% of the cases), and it is less frequently found in poorly differentiated or anaplastic thyroid cancers. BRAF mutations are identified in a prevalence of approximately 60% of classic PTC, 80% of tall cell variant PTC, and only 10% of follicular variant PTC. A multicenter study demonstrated a significant association of BRAF V600E with poor clinic-pathological outcomes of PTC not confirmed in other studies. BRAF mutations have been associated with reduced expression of the sodium iodine symporter, resulting in total or partial loss of iodine uptake.
The K601E point mutation has been also described in thyroid cancer but it is less common.
BRAF protein can be activated also by a paracentric inversion of chromosome 7, resulting in the in-frame fusion between exons 1 and 8 of the A kinase (PRKA) anchor protein 9 (AKAP9) gene and exons 9 and 18 of BRAF. Normally, AKAP9 binds to type II regulatory subunits of protein kinase A contributing to maintain the integrity of the Golgi apparatus. In the fusion protein, the autoinhibitory aminoterminal part of BRAF is lost and the chimeric product contains only the BRAF kinase domain, thus resulting in an elevated kinase activity. This rearrangement has been found in approximately 11% of PTC after radiation exposure and only in 1% of PTC with no history of radiation.
hTERT
Telomerase is a reverse transcriptase enzyme that maintains chromosome ends by addition of DNA sequence repeat TTAGGG to the 3’ end of DNA strand. The enzyme is composed of a telomerase RNA component (TERC) that binds to the 3’ overhang of telomeric DNA and serves as a template for the addition of repeats, a protein component called telomerase reverse transcriptase (hTERT) and specific accessory proteins. TERC maps on chromosome 3q26.2 and is a 451 nucleotide-long noncoding RNA. hTERT maps on chromosome 5p15.33 and is characterized by eight different transcripts, four of them coding for 1132, 1069, 807, and 1120 a.a. length proteins. According to mutational and bioinformatics studies, hTERT is formed by three main structural elements: (1) a long N-terminal extension containing conserved DNA and RNA-binding domains; (2) a central catalytic domain with the reverse transcriptase activity; and (3) a short C-terminal domain. In embryos during development, telomerase activity is required from the beginning of meiosis and is strongly activated at the morula/blastocyst transition. Its activity and expression are elevated in all tissues and start to be downregulated when tissues differentiate. On the contrary, normal somatic cells do not express telomerase and have a finite replicative capacity regulated by telomere length.
Mutations of the hTERT promoter, encoding for the catalytic domain of telomerase, have been recently described in differentiated thyroid cancer, mainly in poorly differentiated, more aggressive tumors and in anaplastic thyroid carcinoma.
Other genetic alterations
Other rare mutations, such as TP53, β-catenin, PIK3CA, AKT1, isocitrate dehydrogenase 1 (IDH1), and epidermal growth factor receptor (EGFR) mutations, have been recently described, mostly in poorly differentiated and anaplastic cancer.
TP53
This protein, also known as cellular tumor antigen P53, is encoded by a gene located at 17p13.1 and responds to diverse cellular stresses to regulate expression of target genes, thereby inducing cell cycle arrest, apoptosis, senescence, DNA repair, or changes in metabolism. Its regulation of cell cycle is mediated by controlling a set of genes required for this process such as an inhibitor of cyclin-dependent kinases. When cell conditions do not allow DNA damage to repair, TP53 induces apoptosis either by stimulation of BAX and FAS antigen expression, or by repression of Bcl-2 expression. Moreover, cooperation with mitochondrial PPIF is involved in activating oxidative stress-induced necrosis.
β-Catenin
Catenin (cadherin-associated protein), beta 1, 88 kDa, also known as the β-catenin gene, is located at 3p22.1. The encoded protein is part of a complex of proteins that constitute adherens junctions, which are required for the creation and maintenance of epithelial cell layers by regulating cell growth and adhesion between cells. In addition, the protein also anchors the actin cytoskeleton and may be responsible for transmitting the contact inhibition signal that causes cells to stop dividing once the epithelial sheet is complete. Finally, this protein acts as a coactivator for transcription factors of the TCF/LEF family, leading to activate Wnt responsive genes.
PI3KCA
Phosphatidylinositol 4,5-bisphosphate 3-kinase catalytic subunit alpha gene is located at 3q26.3. The encoded protein belongs to a family of lipid kinases capable of phosphorylating the 3′OH of the inositol ring of phosphoinositides. They contribute to coordinate a different range of cell functions including proliferation, cell survival, degranulation, vesicular trafficking, and cell migration through activation of AKT1, RhoA, PDK1, PKC, and other factors.
AKT1
AKT1 (gene at 14q32.33) is one of three closely related serine/threonine-protein kinases (AKT1, AKT2, and AKT3) that regulate many processes including metabolism, proliferation, cell survival, growth, and angiogenesis by phosphorylating a range of downstream substrates in response to growth factor stimulation (i.e., EGF, IGF). AKT is responsible of the regulation of glucose uptake by mediating insulin-induced translocation of the SLC2A4/GLUT4 glucose transporter to the cell surface. It also regulates the storage of glucose in the form of glycogen. AKT promotes cell survival via the phosphorylation of the apoptosis signal-related kinase MAP3K5. Phosphorylation of “Ser-83” decreases MAP3K5 kinase activity stimulated by oxidative stress and thereby prevents apoptosis. AKT has an important role in the regulation of NF-kappa-B-dependent gene transcription and positively regulates the activity of the cyclic AMP (cAMP)-response element binding protein (CREB1). The phosphorylation of CREB1 induces the binding of accessory proteins that are necessary for the transcription of pro-survival genes.
IDH1
Isocitrate dehydrogenases (2q34) catalyze the oxidative decarboxylation of isocitrate to 2-oxoglutarate to produce alpha-ketoglutarate (α-KG), playing a key role in the Krebs cycle. The enzyme activity is dependent on nicotinamide adenine dinucleotide phosphate (NADP + ) and the biochemical reaction, catalyzed by IDH, leads to the production of NADPH, which plays an important role in the cellular control of oxidative damage. IDH1 mutations were found to be exclusively in codon 132.
Epidermal Growth Factor Receptor
The EGFR gene (7p11.2) encodes for a transmembrane glycoprotein that functions as a receptor for members of the epidermal growth factor family. Binding of the protein to its ligand induces receptor dimerization and tyrosine autophosphorylation and leads to cell proliferation. Known ligands include EGF, TGF-alpha, amphiregulin, epigen, betacellulin, epiregulin, and heparin-binding EGF. The activated receptor recruits adapter proteins like GRB2, which, in turn, activates important downstream signaling cascades including the RAS-RAF-MEK-ERK, PI3 kinase-AKT, PLC-gamma-PKC, STATs, and NF-kappa-B. EGFR also directly phosphorylates other proteins like RGS16, triggering its GTPase activity and probably coupling the EGF receptor signaling to the G protein-coupled receptor signaling.
In summary, it is now evident that the most important driver of genetic events of papillary and follicular thyroid cancer, respectively, occur in the MAPK and PI3K/AKT pathways. It is also evident that mutations seen in thyroid cancer are almost always mutually exclusive. In the rare cases where multiple mutations accumulate, the cancer phenotype is usually more aggressive.
A summary of the most frequent mutations found in thyroid cancer is reported in Table 10.1 .
Oncogene mutations | Rearrangements |
---|---|
BRAF (V600E) | RET/PTC (1, 3, others) |
RAS (N, H, K) | PAX8/PPARγ |
hTERT | NTRK |
PIK3CA | BRAF/AKAP9 |
PTEN | ALK |
AKT1 | |
TP53 | |
CTNNB1 | |
GNAS |
Application of molecular findings to the clinical diagnosis of thyroid cancer
The frequency of thyroid nodules in clinical practice has been increasing year by year after the introduction of neck ultrasound in clinical practice. Fortunately, most of the nodules are benign, with only 5–7% being malignant. Thyroid nodules are not a unique disease but are the clinical manifestation of several different thyroid diseases. They may be single or multiple and may be found in the context of a normal gland or a diffuse goiter. In multinodular goiter, one of the nodules may become clinically dominant in terms of growth, dimension, and functional character. The main problem posed by the discovery of a thyroid nodule is the distinction between its benign or malignant nature and, consequently, its appropriate treatment. Several clinical and instrumental findings may suggest the nature of the nodule, but none of them is sufficient for a diagnosis. Up to now, the gold standard for the differential diagnosis of thyroid nodules is represented by fine needle aspiration cytology (FNAC). Compared to pre-FNAC years, in centers that use FNAC results for treatment decision making, there has been a 35–75% reduction in the number of patients sent to surgery, and a two– to threefold increase in the percentage of cancers found among surgical nodule specimens. In addition, the result of FNAC has been better planning of the surgical intervention. However, despite its high specificity and sensitivity, FNAC is not diagnostic in about 20–30% of cases due to the presence of an indeterminate category of nodules, the so-called follicular proliferation, in which the cell features are not distinguishable in benign or malignant. To overcome this limitation, the search of genetic profiles associated to thyroid nodules has been proposed with promising results. So far, two possible approaches have been implemented: search for known oncogene mutations or search for benign gene profiling.
Search of Oncogene Mutations
This approach is based on the rationale that cumulating all the mutations that occur in thyroid cancer (point mutations and rearrangements), at least one mutation is found in nearly 90% of thyroid cancers. In addition, some of the most frequent mutations are 100% specific for malignancy with no false positive results. BRAF mutation analysis has high specificity (almost 100%) but low sensitivity, being present in only 40–45% of PTC. To increase the sensitivity, a large panel of oncogenes should be included in the analysis. Several studies have shown the validity of using a panel of oncogenes including at least BRAF, RET/PTC, PAX8/PPAR, and hTERT mutations with the possible addition of TRK rearrangement. When one of them is present in the nodule specimen, a diagnosis of malignancy is almost certain at final histology, assuming that the analysis is performed in a clinical laboratory and after validation of detection techniques. On the basis of the high probability of cancer in nodules carrying one of the above mutations, these patients should be treated with total thyroidectomy. This will avoid the repeat of FNA and eliminate the need for a two-step surgery (lobectomy followed by thyroidectomy). At variance, missense mutations of RAS have a 74–87% positive predictive value for cancer, but in the remaining cases may be associated with a diagnosis of follicular adenoma. These results are only apparently false positive, if one considers that follicular adenomas are most likely premalignant lesions, and thus an indication for surgery based on positive mutational status might be considered as true positive. When no mutations are found in FNA material, the risk that the nodule is malignant is reduced to 3–5%, but is not completely eliminated. Patients are sent for follow-up, FNA is repeated, and, if the diagnosis of indeterminate nodule remains, lobectomy can be offered. To measure these mutations numerous “homemade” methods are available from end-point polymerase chain reaction (PCR) (including ASO-PCR for BRAFV600E mutation and nested PCR for rearrangements) to real time or high resolution melting PCR. Screening with denaturing high-pressure liquid chromatography and final Sanger sequencing (or better pyrosequencing) are also recommended. In addition, several commercial kits are now offered by companies to assay all RAS and BRAF point mutations by real-time PCR using a multiplex approach whereas the “AmoyDx™ RET 14 Rearrangements Detection Kit” (developed by AmoyDx, China, with a contract agreement with AstraZeneca) is the only commercial assay for RET/PTC rearrangements detection with a limitation for research use only. A commercial panel including BRAF, RAS, PI3KA mutations, and RET/PTC, PAX8/ PPARγ rearrangements available in the United States (except for New York and Florida states) is the ThyGenX by Interpace Diagnostics. Specimen collection kits may be ordered by physicians or other authorized health care professionals and, after sample collection, sent to the factory for analysis.
A more comprehensive analysis is offered by the next-generation sequencing (NGS) approach, which is able to interrogate multiple genes simultaneously in a cost-effective way with high sensitivity and working with low input of starting material (10 ng). A commercial NGS panel for thyroid cancer is represented by Thyroseq ® (UPMC Life Changing Medicine), which works on DNA or RNA isolated from FNA material collected in preservative solution, fixed FNA specimens, or formalin-fixed, paraffin-embedded (FFPE) tumor tissue. The analytical sensitivity is 3–5% of mutant alleles for detection of mutations and 1% for detection of gene fusions. Thyroseq is performed by the MGP Laboratory of the University of Pittsburgh Medical Center upon requirement of dedicated tubes for sample collection.
Recently, driver mutations were uncovered in 95% of PTC, and modern techniques will probably lead to even more accurate diagnoses. By extending the molecular analysis to a panel of point mutations in 13 genes and 42 types of gene fusions using a proprietary sequencer and NGS, the risk of malignancy is further decreased if no mutations are detected.
Based on the current experience, it is apparent that mutational analysis of a nodule is particularly useful in case of indeterminate lesions. The results of FNAC integrated with the mutational screening of the nodules may direct the therapeutic strategy as reported in Table 10.2 .
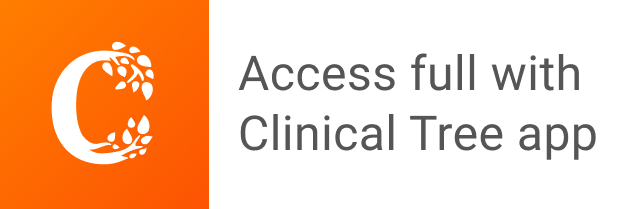