Fig. 41.1
Activating mutations in K-Ras occurring early on during pancreatic cancer development lead to higher proliferating rates of affected cells. While this may trigger the cell surveillance system that may cause cell growth arrest and apoptosis or senescence, the additional loss of expression of p16INK4A and/or mutation of TP53 allow the higher proliferating potential of the cells and the progression toward malignancy.
Table 41.1
Candidate biomarkers for cancer detection, risk assessment, and diagnosis
Type/marker name | Cancer type (organ) | Biospecimen | Utility | Reference |
---|---|---|---|---|
Epigenetic VHL p16INK4a TIMP3 Gene methylation panel | Kidney Pancreas Colon Lung | Tissue Pancreatic fluid Tissue Sputum, Plasma | Risk assessment Risk assessment Disease recurrence Diagnosis | [97] [18] [98] [98] |
Genetic K-Ras (mutation, amplification) Bethesda microsatellite panel Microsatellite assay (MSA) FISH panel | Pancreas Colon (HNPCC) Bladder Esophagus | Pancreatic/bile fluids Tissue Urine Tissue | Early detection Diagnosis Early detection Disease prediction | [99] [97] [98] [98] |
Genomic OncotypeDx Mammaprint | Breast Breast | Tissue Tissue | Disease prediction Disease prediction | [70] [80] |
Proteomic Des-γ-carboxyprothrombin (DCP) Alpha-fetoprotein (AFP)-L3 MALDI 6-peak profile Yale 6-protein marker panel COX-2/Ki67/p16INK4a MMP1/CEACAM6/HYAL1 Mucin protein panel assay | Liver Liver Liver Ovary Breast Breast Pancreas | Serum Serum Serum Serum Tissue Tissue Serum | Early detection Early detection Early detection Early detection Disease prediction Disease prediction Early detection | [94] [94] [98] [95] [84] [98] |
41.3 Early Detection Versus Diagnosis
A cancer biomarker is defined as any molecular or biochemical alteration that can be measured and effectively used in a clinical scenario for cancer detection, diagnosis, prognosis, and prediction of therapeutic response. Cancer detection usually involves clinical testing of asymptomatic healthy subjects as in population screening or following stratified high-risk cohorts for the presence of preclinical/early disease. It has several implications in the clinical context, such as enabling further investigation to determine the extent of disease, or taking measures to contain the initial disease by surgical removal, limiting therapeutic treatment, or adapting preventive care. Ideally, a cancer biomarker for detection is present in easily accessible body fluids such as saliva, blood, urine, upper digestive tract effusion, and the material that can be extracted by fine needle aspirates and other minimally invasive procedures. It enables easy screening of a larger population without requiring extensive training and clinical expertise. However, biomarkers for diagnosis need to provide extensive information about the tumor and its microenvironment. For this reason, tissue biopsies and other invasive procedures are also used when necessary to enable critical decisions.
To be useful for detection, a cancer biomarker ideally should be able to distinguish normal individuals from cancer patients with 100 % accuracy. Sensitivity and specificity determine how accurately a biomarker can predict the presence of a disease and its ultimate utility in clinical practice. Sensitivity is defined as the ability of the biomarker to detect the cancer cases (true positive); while specificity is defined as the ability of the marker to identify the healthy cases (true negative). A useful biomarker should be able to detect cancer with high precision, allow early detection of the premalignant lesions and ultimately reduce mortality due to cancer. Unfortunately, most of the current biomarkers do not have sufficient diagnostic power to be clinically useful if used alone. The widely used clinical marker prostate-specific antigen (PSA) has only 70 % sensitivity and 59–97 % specificity [26–28]. When used in population screening, PSA, due to its low specificity, leads to many false positives and triggers unnecessary biopsies and clinical follow-up. CA-125, the biomarker for the detection of ovarian cancer, has only 70 % sensitivity and 87 % specificity and is not currently recommended for population screening [29–32].
In contrast, diagnosis of cancer requires more confirmatory tests with high precision and sensitivity to enable more critical decisions, such as surgical removal of vital organs, decisions related to suitable chemotherapy, preserving unaffected organs and follow-up. Moreover, it is important to differentiate different types of cancers that affect the same organ to enable additional diagnostic and efficient treatment strategies. BRCA1 and BRCA2 mutations affect the incidence of genetically predisposed breast cancers. Genetic screening of breast cancer patients for these mutations is complex and time-consuming owing to the large size of both genes. It is desirable to have more understanding of both the phenotype and the morphological and molecular characteristics of the tumors underlying these mutations. BRCA1-associated tumors are usually poorly differentiated, infiltrating ductal carcinomas and show frequent morphological features of atypical medullary carcinomas. BRCA2-associated tumors tend to be of higher grade than sporadic cancers. Additionally, BRCA1 tumors are usually estrogen receptor and progesterone receptor negative and p53 positive, whereas such changes are not seen in BRCA-2-associated tumors. Both BRCA1-associated and BRCA2-associated breast tumors show a low frequency of Her-2 overexpression compared to sporadic cancers, in addition to various other molecular abnormalities [11]. Such information can be especially useful in making decisions in appropriate screening and therapeutic choices. However, the current imaging modalities only provide spatial resolution of tumors, but not their molecular composition. As such, there is a continuing need for multiple molecular markers to enable accurate detection and diagnosis of the disease.
41.4 Molecular Biomarkers of Cancer
The biology of carcinogenesis provides a large window of opportunity to study the range of molecular events that can provide information on malignant transformation. Over the last 30 years, there has been a tremendous explosion in the understanding of cancer biology. For example, it has been well characterized that Ras and Raf mutations and alterations in downstream MAP kinase pathways frequently form the early events in malignant transformation and have been explored as potential anticancer targets in a variety of cancers [33]. Epidermal growth factor receptor (EGFR) mutations have been implicated in the progression of breast, lung, and other cancers. Epigenetic changes and P53 mutations have been indicators of late events of progression [33–35]. While many of the alterations in these pathways could provide molecular signatures that oncologists can reliably use in the detection and diagnosis of cancer, none of them have actually translated well into clinical application. One of the main problems in molecular diagnosis of cancer is that it is a cellular proliferation disease and many of the cancer-related proteins and changes are part of normal pathophysiological phenomena and not specific to cancer. No abnormal molecular changes are effectively detected that indicate malignant transformation. Many of these events are viewed as part of the body’s normal inflammatory responses. For example, it has been demonstrated that many of the initial changes are usually confined to the tumor microenvironment, making them inaccessible for noninvasive molecular diagnostic approaches [36]. The magnitude of the malignant changes associated with minute tumors by and large go undetected mainly because these changes are very small compared to variations in normal cellular and physiological processes. Confounding this problem is that most of the molecular detection approaches lack sufficient analytical sensitivity to detect such subtle changes. The detection sensitivity of currently used ELISA and other clinical assays is 1–2 pg/ml, which fails to detect molecular markers associated with cancer that are secreted at much lower levels (fg/ml) [37–40]. Detection systems with much lower detection limits (in Pico- and Femto-molar concentrations) are needed to detect such abnormal proteins.
41.5 New Technologies for Detection and Diagnosis Marker Discovery
Genome-wide DNA sequence analysis has led to a surge in the enthusiasm for the application of distinct high-throughput approaches to analyze multiple biomolecules giving rise to novel omic technologies. Using these technologies, it is now possible to analyze multiple molecular changes in DNA (genomics), RNA (transcriptomics), proteins (proteomics), or metabolites (metabolomics) in a limited sample size in a single platform, thereby saving time, efforts, and costs.
Current cancer research has taken a multidisciplinary approach to address the gaps in the translation of cancer molecular biomarkers into the clinic. This approach may lead to the identification of novel biomarkers that conventional approaches have long failed. High-throughput molecular analytical techniques combined with the latest tissue acquisition techniques, such as laser-aided microdissection enable global molecular profiling with very high precision. Both targeted and untargeted approaches are employed giving rise to information on either known molecular species or molecular profiles, such as in mass spectrometry. The resulting data are analyzed using state-of-the-art computational analytical tools for pattern recognition allowing the differential diagnosis of cancer. It is generally unnecessary to identify the molecular nature of the peaks (black box approach). With computational methods, it is possible to integrate the information from diverse markers to improve their clinical sensitivity and specificity for cancer detection and diagnosis. This has opened the door to a novel paradigm of cancer diagnosis in which a single marker for a single disease is no longer the norm. Instead, multiple markers for a single disease are the mode of choice. It is assumed that such a strategy allows accurate measurement of unique biological processes measured simultaneously and integrated for improved diagnosis.
41.6 Role of Specimens in Biomarker Development
Most of the molecular analysis approaches rely on the availability of freshly collected tissues as compared to fixed tissue and archived specimens. Some of the molecular approaches demand highly defined and precise cellular species. The introduction of laser scanning capture microdissection (LSCM) techniques into clinical specimen collection has improved the quality of the specimens for analysis. The need for immediate sample processing, fresh-frozen tissues, and concomitant specimen storage conditions, and the effect of the length of storage time make the molecular diagnostic approaches difficult to adapt in clinical practice. These issues undermine the utilization of existing repositories for biomarker development. However, some recent development efforts focused on the utilization of fixed tissues by high-throughput molecular approaches are improving this situation. The collection of specimens for molecular analysis, such as nipple aspirate fluid and ductal lavage for breast cancer detection and pancreatic effusion fluid for pancreatic cancer detection, poses further challenges in molecular diagnostics, and the development of special collection methods is needed.
41.7 High-Throughput Analysis and Biomarker Development
One of the main issues in high-throughput technological analysis is analytical sensitivity. Depending on the sensitivity of the technology employed, the numbers of molecular species resolved can vary widely. For example, 2D gel electrophoresis has a limited resolution of only 200–300 proteins per analysis and mainly focuses on the resolution of highly abundant proteins. More advanced mass spectrometric analyses, however, allow the resolution of hundreds to thousands of proteins ranging from MW 2000 to 30,000. As such, it may not always be possible to resolve both high-abundant and low-abundant proteins on the same technology platforms but it is usually difficult to compare proteins resolved on different platforms. It is possible to identify some of the low-abundant proteins (functional peptidomics), using more advanced mass spectrometric techniques combined with time-of-flight (TOF) techniques, and exoprotease digestion techniques [41]. Mass spectrometric techniques routinely suffer from inconsistencies in reproducibility mainly because of the nature of instrumentation, mass calibration shifts and factors related to baseline correction in mass spectrometric analysis [42, 43]. Another confounding factor in reproducibility is the batch variations in protein chips; it is normally difficult to overlay the sets of features from different runs of the same specimens and to validate these molecular features as biomarkers.
High-throughput approaches , such as mass spectrometry, yield patterns and profiles that are subjected to computational analyses to simplify the dimensionality of the data and to identify meaningful patterns that may be clinically significant. Two major types of computational approaches, namely supervised and unsupervised methods, are applied to analyze the data. In the supervised methods, the information is organized in a predetermined fashion as to which molecular changes are expected to group together and the resulting patterns are analyzed collectively for their ability to differentiate cancers. When applying supervised approaches, it is difficult to analyze the patterns without established classification rules. Depending on the size of the training set, the test results give diverse patterns that may not be reproducible. With unsupervised methods, the data are grouped based on their inherent expression patterns and the resulting profiles are tested for their ability to group together and predict the outcome. Unsupervised approaches rely on imposition of algorithms that tend to fault depending on the method. Thus, the method of data analysis plays an important role in biomarker development. One of the confounding aspects with high-dimensional data analysis is the signal-to-noise ratio. Many genes undergo changes in expression that are not necessarily specific and related to cancer. These changes are recorded by microarray and other high-throughput approaches, thereby resulting into complex patterns and enormous volumes of data and leading to erroneous over-fitting of the data.
Another critical factor in the application of high-throughput technologies in biomarker development is that the platforms for discovery and development may not be the same as for clinical application, where more directed, simpler, and specific tests are needed. Typically, more than one approach can be applied to detect a molecular marker. For example, three molecular tests for human epidermal growth factor receptor-2 (Her-2/neu) oncogene expression have been approved by the Food and Drug Administration (FDA): one to monitor the treatment response of breast cancer with herceptin, a second to determine the sensitivity to doxorubicin therapy, and the third for the early detection of recurrence and to determine the prognosis of breast cancer [44–46]. Her-2 expression can be detected by various methods at the protein level (by immunohistochemistry; IHC), at the DNA level [using fluorescence in situ hybridization (FISH) analysis and polymerase chain reaction (PCR) amplification] or at the messenger RNA level. The FDA-approved tests include a FISH-based molecular assay and two antibody-based assays. However, a number of antibodies with varied sensitivity are commercially available, which together with kit to kit variability they make the detection of Her-2 a complicated task with many false-positive cases and misleading results. While FISH analysis is very sensitive, its high cost and marginally superior results make it less attractive as a routine monitoring tool [46]. Thus, the choice of the test method and its application depends on the analytical sensitivity and the cost-effectiveness.
The molecular profiles or signatures that arise from mass spectrometric and other high-throughput approaches may have clinical significance and could be successfully applied in the clinic if they have high sensitivity and specificity (even without prior knowledge of the molecular species contributing to the signatures). However, the negative results are difficult to interpret; hence, the absence of information on the peaks can often lead to inconclusive results. These molecules therefore should be further characterized using more specific analytical methods such as southern, northern, and western analyses or quantitative polymerase chain reaction (qPCR) and IHC, which can allow easier application of the molecular tests in a clinical setting [47].
Approaches to detect LMW proteins include the use of nanoparticles conjugated to molecular baits. These enable selective LMW protein binding and release on harvest platforms with nanoparticles such as nanobarcodes with distinct physicochemical properties [48].
One of the impediments in cancer biomarker development is that the tumor-specific proteins and cytokines are secreted at very low concentrations below the analytical sensitive range of most clinical methods. The real challenge is to identify such low abundant proteins that can provide critical information on the nature of a tumor. A variety of approaches are applied to detect such proteins either on sandwich arrays, where a pair of antibodies is used to detect each protein, or a label-based system, where target proteins are labeled to enable detection after they bind to the arrays. These approaches are highly sensitive and specific and assays are generally easy to develop [49, 50]. There are also other approaches that may significantly improve the sensitivity, accuracy, and reproducibility [50]. These include the two-color rolling-circle amplification antibody arrays, which amplify the signals from labeled proteins. Such methods allow detection of multiple proteins from two different sets of samples simultaneously. Two sets of samples are covalently labeled with biotin and digoxigenin individually and incubated with antibody arrays. The immobilized labeled proteins are detected using antibodies against biotin and digoxigenin that are covalently conjugated to two separate primers. The primers are amplified by complementary circular DNAs tagged to two different colors (cy5 and cy3) by DNA polymerase. This results in signal amplification in two colors for two different sets of proteins. These approaches allow acquisition of expression profiles of multiple proteins from diverse samples and require very small biological specimens with minimal processing, making them attractive analytical tools. Although still in the developmental stage, these tools are beginning to show promising results when applied to clinical specimens [50].
Host immune systems play a critical role in surveying infectious and foreign bodies, including tumor antigens, and responding by eliciting antibodies, thereby paving the way for novel detection strategies for early detection of cancers. Autoantibodies are promising tools for detecting cancers as they are elicited in response to low concentrations of circulating tumor antigens; and the production of a large repertoire of antibodies makes the detection highly sensitive. Unlike tumor antigens, which are usually degraded by proteases, the antibody response persists for a long time even after the antigen has been removed from the circulation. They are more stable with a relatively long half-life in circulation. Assorted targeted antibody array approaches are attempting to identify cancer-specific autoantibodies that have promise for cancer detection, diagnosis, and therapeutics. These approaches make use of antigen libraries created on a variety of biological vectors such as phages, yeast, and others created from cancer cell lines, or by expressing protein in situ using DNA vectors and in vitro translation methods [51, 52]. The protein arrays are challenged with sera from healthy individuals and cancer patients to identify cancer-specific autoantibodies that interact with tumor antigens present on the arrays. Although the field is still emerging, growing evidence indicates that this approach may have important implications in cancer detection and diagnosis [51, 52]. Other emerging biomarker discovery modalities include the application of molecular species-specific flow cytometry to study pathological signal networks at the single cell level. Danna et al have used this approach to study molecular signaling alterations in acute myeloid leukemia [53].
MicroRNAs are small non-coding highly conserved RNAs that are derived from pre-miRNA precursors by cytosolic RNase action. They play an important role in diverse cellular functions including proliferation, differentiation, and death. Currently, more than 400 miRNAs have been identified. The relatively small numbers of miRNAs and their role in cellular functions make them promising candidates as biomarkers for diagnosis and detection. Despite the fact that the miRNA field is still in its infancy, evidence suggests miRNAs can provide critical information on the diagnosis, prognosis, and therapeutic targets of cancer [54]. Using miRNA microarray analysis, Bloomston et al identified several miRNAs that are differentially expressed among pancreatic adenocarcinoma, benign pancreatic tissue, chronic pancreatitis, and normal pancreas [55]. Using the Predictive Analysis of Microarrays method, they identified a subset of 21 miRNAs overexpressed and four miRNAs underexpressed that could discriminate pancreatic cancers from normal pancreatic tissue with 90 % accuracy. In the same study, they identified a set of 15 miRNAs overexpressed and eight miRNAs underexpressed which could identify pancreatitis from pancreatic cancers with 93 % accuracy. A set of 15 overexpressed miRNAs and two underexpressed miRNAs could differentiate pancreatitis from normal pancreas. These early results need further study, but these studies demonstrate the potential role of miRNAs as candidate diagnostic markers. miRNAs are being explored in many cancers for their diagnostic ability [54].
Other omic technologies that hold promise for the development of novel cancer biomarkers are metabolomics, the study of small molecules or molecular profiles in tissues or body fluids. Metabolomics broadly applies to nuclear magnetic resonance (NMR) and mass spectrometric technologies to measure a variety of metabolites or their profiles, which leads to enormous amounts of data. The relatively small number of metabolites compared to the number of proteins or genes present in the human body makes them attractive candidates for disease detection, diagnosis, and other clinical applications. However, the metabolome or metabolic profiles of a physiological system is dynamic and subject to influences such as environmental, pharmacological exposure, temporal, dietary, physiological, age, sex, and genetic predisposition. The technology has great promise and its potential is only beginning to be explored in cancer research. Recently, metabolomics technologies were applied to the differential diagnosis of ovarian cancer using differences in spectral patterns of healthy and affected women [56]. In this study, sera from 12 women with benign ovarian cysts, 38 ovarian cancer patients, 19 pre-menopausal, and 32 post-menopausal healthy women was subjected to 1H-NMR spectroscopy and the resulting data were analyzed using both supervised and unsupervised methods. Demonstrating the promise it holds for future molecular diagnosis, researchers were able to identify a pattern that could discriminate between ovarian cancer and healthy subjects. Moreover, these patterns allowed discrimination between benign cases and ovarian cancer patients. Using fine needle aspirate biopsies from 140 patients with breast lesions analyzed by 1H-NMR spectroscopy and pattern recognition analysis, Mountford et al were able to classify benign cases from malignant cases with 93 % sensitivity and 92 % specificity [57].
41.8 Molecular Imaging in Cancer Detection and Diagnosis
Functional magnetic resonance (MR) techniques such as dynamic contrast-enhanced MR imaging (DCE-MRI) and magnetic resonance spectroscopic imaging (MRSI) combine the virtues of conventional imaging with molecular profiling. Besides the spatio-temporal resolution of tumors, these enable additional novel molecular information on tumor metabolism, and provide more sensitive and accurate strategies for molecular diagnosis. Using this strategy, van Dorsten et al have shown that the diagnostic sensitivity of prostate tumor regions can be significantly enhanced by adding metabolic profile information to the quantitative DCE-MRI [58]. It was shown that the ratio of (choline + creatine)/citrate was elevated in prostate cancer compared to healthy zones peripheral to the tumor and in the central gland. A ratio above 0.68 is a reliable indicator of cancer in determining the cancer-affected regions. Such an approach is feasible in clinical practice and has excellent clinical implications. Positron emission tomography (PET) imaging is another modality that has been approved by the FDA to determine cancer staging. It provides useful information on spatio-temporal resolution of the tumors. With the aid of specialized metabolic analogs, it is possible to visualize the tumor metabolism to distinguish between indolent and malignant cancers and normal tissues. The most frequently used PET agent is [18F] fluorodeoxyglucose (FDG), a glucose analog taken up by highly metabolizing tumor cells, which allows the study of tumor metabolism. Many contrast agents have been approved by FDA for clinical applications that are used in molecular imaging. This approach has been approved for tumor staging of several cancers including breast, lung, and colon [59–62].
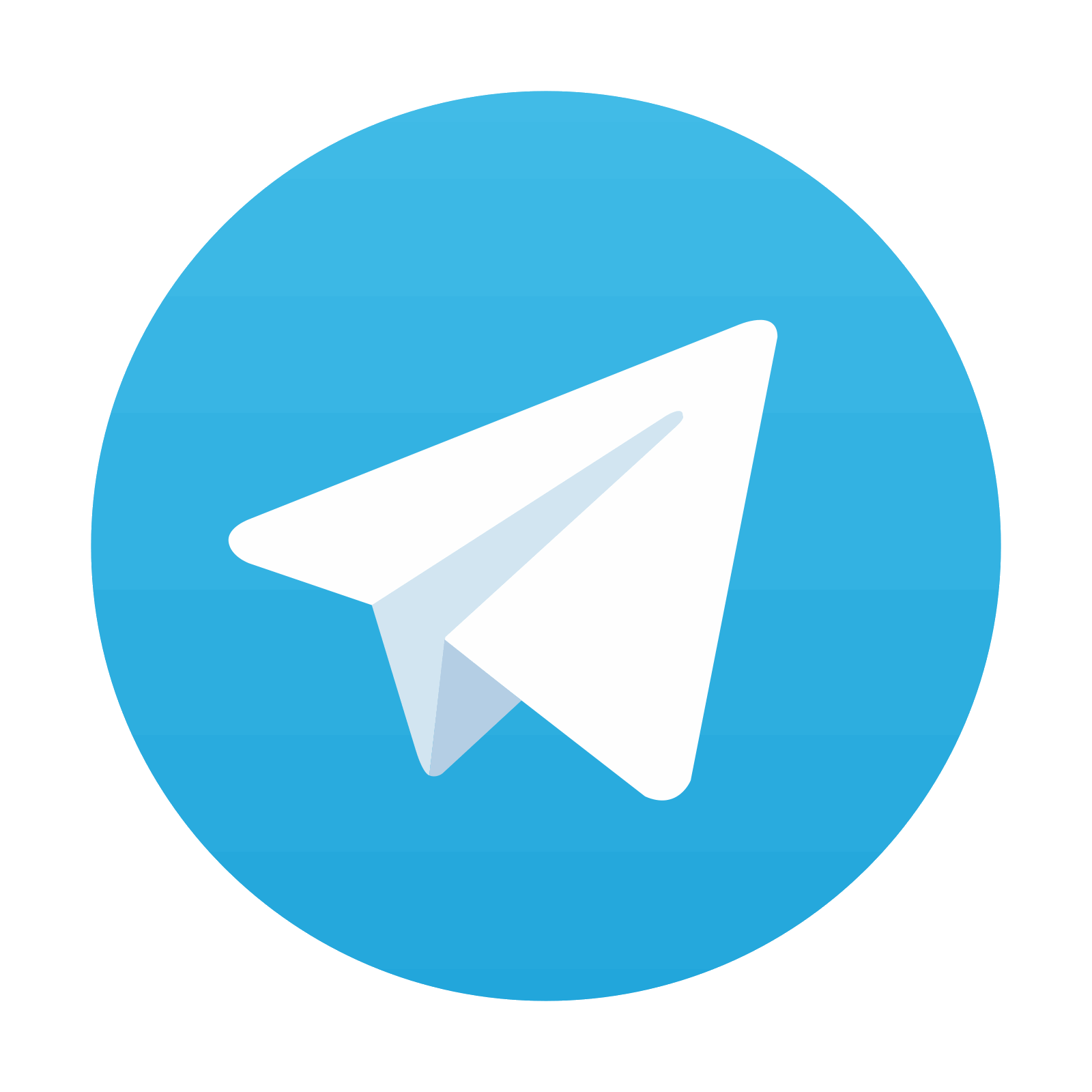
Stay updated, free articles. Join our Telegram channel
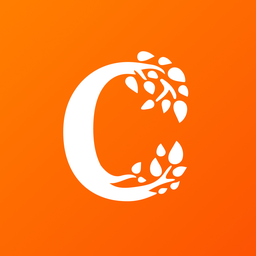
Full access? Get Clinical Tree
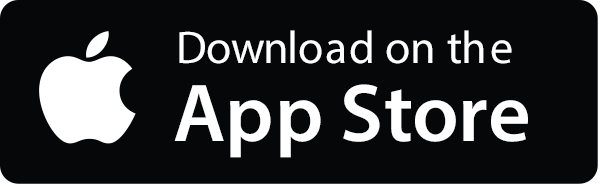
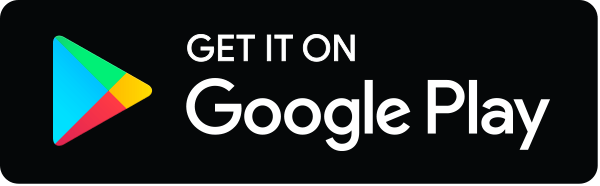