2.1 Introduction
Our planet Earth is estimated to be around 4.5 billion years old. Life on this planet is thought to have emerged at least 3.8 billion years ago. The emergence of entirely new species, and the extinction of many others, has been driven by natural selection. So, we believe, has the immune system.
Each new species that evolves represents a new ecological niche that can be used by other species (e.g. as food or shelter, or as a vehicle for transmission of their genes) and which can be considered as parasites in the most general sense. The emergence of these species, both the hosts and the parasites, has been driven by natural selection. Parasites and their hosts are involved in an evolutionary arms race. In most cases it is not to the parasite’s advantage to kill its host because this would prevent spreading of parasite genes. If, however, the host evolves new defence mechanisms to prevent or limit infection, any parasite that evolves ways of overcoming these mechanisms will have a selective advantage and will reproduce more successfully. Reciprocally, but generally much more slowly, a host that mutates to resist parasites more effectively will also have a selective advantage. Thus, it is host–parasite interactions that drive the evolution of immune defence mechanisms in the host.
Viruses and microscopic organisms such as bacteria and small protozoa, that may collectively be termed microbes, evolve much faster than their mammalian hosts. If a microbe mutates so that it is “invisible” to the host’s immune system it would take many generations before the host could evolve effective defence. If, however, the host had evolved anticipatory mechanisms that could recognize any variants that might occur in a microbe, it would be well placed to cope with many mutants. This is precisely what the adaptive immune system in mammals has achieved by randomly generating lymphocytes that express a vast diversity of antigen receptors.
In this chapter we examine immunity to infection in mammals. We start by introducing the different types of organism that can infect us and explain that, while the vast majority of these organisms do not cause disease, some of them, the pathogens, can cause serious disease and death in normal individuals (Section 2.2). We note that we have mutually beneficial relationships with the commensal bacteria that continuously inhabit our bodies. We introduce the concept that defects in immunity, the immunodeficiencies, can result in opportunistic infections by microbes that are otherwise rapidly eliminated by those with functioning immune systems. We emphasize that the types of infection that occur in an immunodeficient host provide evidence for the normal functioning of that component in immunity.
We then explain the function of each major component of immunity in defence against infection, using studies of natural human defects and the responses of genetically modified mice as evidence for these functions (Section 2.3). We next outline how some of the main classes of infectious agent can cause disease and explain how the different components of immunity, considered in isolation earlier, work together to try to eliminate them (Section 2.4). To illustrate the different interactions between hosts and different classes of pathogens we use selected case studies to describe the type of disease that each can cause. We discuss why disease happens, how different mechanisms of immunity interact during different types of response, and how these diseases can be prevented or treated by strategies based on our knowledge of immunity.
Finally, we discuss briefly the vaccines that have successfully eradicated some of infectious diseases, but which are not available for so many others, and outline some new approaches for vaccine design that are being developed for the future (Section 2.5).
2.2 Pathogens and Infectious Disease
2.2.1 What is a Pathogen?
Any given species has very few pathogens. Probably more than 99.9% of all potentially infectious agents are in fact non-pathogenic in normal individuals, and only a few represent opportunistic infectious agents that can cause disease in immunocompromised individuals (Section 2.2.4).
A pathogen is any infectious agent that causes disease. This is, however, too simple a definition. Some pathogens such as rabies virus will virtually always cause disease if they get into the tissues of a mammalian host (e.g. through a bite), but it is highly unlikely that swallowing rabies virus would cause any harm at all. Others, which we often think of as highly pathogenic, such as Mycobacterium tuberculosis (the bacterium that causes tuberculosis), will cause clinical disease only in a minority of healthy people who inhale them. Some, that we think of as normally harmless can cause disease if they are in the wrong place; in other words, they become pathogenic. For example, Escherichia coli, a normal bacterial inhabitant of the large bowel, can cause acute inflammation of the bladder (cystitis) if it gets into the urinary tract.
Other micro-organisms are relatively or completely harmless to most of us, but can cause disease in people with genetic defects of immunity; in other words, primary immunodeficiencies. Pneumocystis jirovecii is a fungal organism very widespread in the environment. We must all be inhaling it at frequent intervals with no harmful outcomes. It is, however, a major cause of illness in patients infected with human immunodeficiency virus (HIV) who progress to a secondary immunodeficiency – acquired immunodeficiency syndrome (AIDS) – as their CD4 T cells are eliminated. The kind of infection that Pneumocystis causes in such cases is called opportunistic. There is an implication of this observation that does not seem to be widely appreciated. Patients with immunodeficiency may become clinically infected by organisms that are harmless to others. So this can only mean that in immunocompetent individuals the immune system is working continually, subclinically, without us being aware at all that defence mechanisms are or have been in action. This suggests that we should radically change our view of the immune system. It is not a system that comes into operation only when we encounter a pathogen; it is a physiological system that is as much a part of normal homeostasis as temperature regulation or the regulation of food intake.
To be a successful pathogen, a microbe must have evolved mechanisms that enable it to evade or avoid host defence (here we use microbe to cover all infectious agents, whatever their size). These mechanisms allow it to infect the host and cause disease (Section 1.2), whereas similar but non-pathogenic agents lacking such mechanisms cannot infect and cause disease. The molecules expressed by pathogens that allow them to infect and survive in their hosts, and which enable them to cause disease, are known as virulence factors. Pathogens use many different mechanisms to subvert immunity and by examining these mechanisms we can learn much about immunity to infection. One authority on vaccinia virus has suggested that we will learn more about immunity to vaccinia from deciphering the vaccinia genome, and hence identifying components the virus might use for subversion or evasion, than by studying immune responses to the virus itself. So what are the different types of pathogen to which humans with otherwise normal immunity are susceptible, and how do they establish infections?
2.2.2 What Types of Pathogen Can Cause Disease?
A remarkable diversity of organisms can be disease causing, or pathogenic, although only a relatively small number of each is pathogenic in any given species such as humans. Perhaps the smallest – although these are not true organisms – are viruses, which include many different types, many probably yet to be discovered. Then there are bacteria, a vast number of different types of single-celled prokaryotic organisms that can live either outside or within the host’s cells (prokaryotes lack a membrane-bound nucleus). Increasing in complexity there are also single-celled and multi cellular eukaryotic yeasts and fungi (eukaryotes possess a membrane-bound nucleus). In addition there is a huge group of organisms classed as true parasites which range in size from microscopic (e.g. protozoa, the malaria parasite) to truly enormous (metazoa, such as helminths such as tapeworms in the gut). We may even have to consider molecules as pathogens if it is the case that diseases such as bovine spongiform encephalopathy (BSE) in humans or scrapie in sheep, are caused by misfolded protein molecules called prions. It is astonishing, but essential, that the immune system has evolved a variety of mechanisms that can potentially attack, and often successfully eradicate, so many different types of infectious agent, differing dramatically in sizes (nanometres to metres) and locations after infection (outside and inside cells, and infecting a variety of different tissues). See Figure 2.1.
Figure 2.1 Major classes of pathogen. Very few potentially infectious agents are pathogenic. A pathogen invades a host to gain shelter, feed or reproduce, and so that it can survive to infect other hosts. (1) The smallest pathogens are viruses, which use the machinery of the host’s cells to reproduce. (2-4) Next in size are bacteria, fungi and single-celled protozoa; some live outside cells, others preferentially live inside cells. (5) The largest parasites are multi-cellular metazoans which are too big to invade cells, but which can live in body cavities, such as intestinal worms. All these can trigger immune responses. Not surprisingly different types of immunity are needed to deal with these different infectious agents.
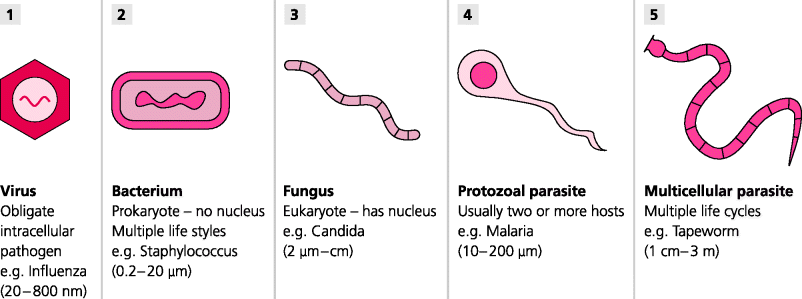
Yet, even if many of these infectious organisms are successfully eradicated in due course, many can still cause disease before this happens; the symptoms we suffer when we have a cold or flu are examples of this type of disease. We will now examine the main classes of infectious agents, some of which are pathogenic, in a little more detail.
2.2.2.1 Viruses
Viruses are subcellular particles that contain nucleic acid (DNA or RNA) in a protein coat. They may or may not have an external lipid envelope derived from host cells. They rely entirely on host cells for their replication. As for other pathogens there are many ways in which they can enter the body; from the air, food or water (e.g. the oro-faecal route for polio), by sexual transmission, or through living vectors (e.g. from an insect bite for yellow fever, or a mammalian bite for rabies). Most or all infectious agents including viruses survive by following a cycle from their reservoirs (natural sources of the agent), through their vectors (which enable them to be transmitted to the hosts through different routes) to their hosts (you or us) and back again. See Figure 2.2.
Figure 2.2 Transmission of infectious diseases. Infectious agents are transmitted to their host from reservoirs, which may include the host itself. The vectors of transmission can be physical (e.g. droplets) or other biological species. Infectious agents can enter the host by different routes, usually by ingestion, inhalation or injection (sexual transmission is also frequent).
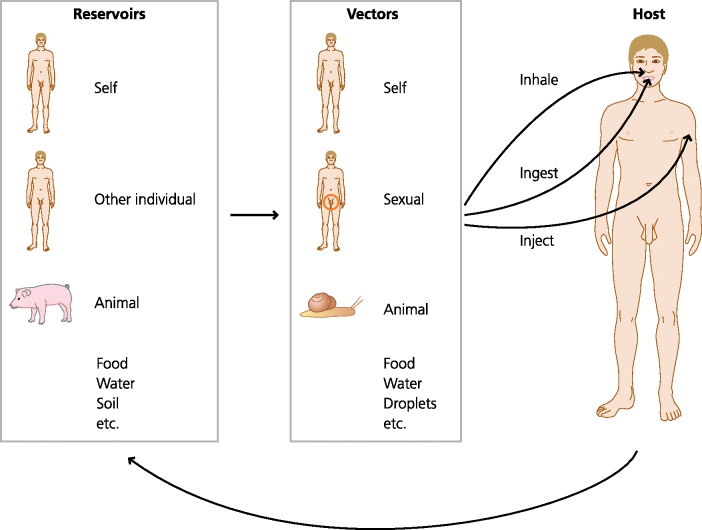
Important barriers to entry of viruses are of course the epithelia – the sheets of cells that cover body surfaces, such as the skin, and the linings of the respiratory and intestinal tracts, and including structures such as secretory glands in the lactating breast and absorptive tubules in the kidney. Some viruses, such as rabies, can bypass the outer epithelial layers of the body through a bite. (In this case the virus even changes the behaviour of its host to increase the chances of transmission to others; it makes them aggressive and more likely to bite.) Most viruses, however, need to infect the first epithelial layer they come to (e.g. in the respiratory tract or intestine). Many viruses, such as influenza, only infect this first epithelial layer. The damage viruses cause to epithelia may then facilitate secondary infections by bacteria; this often happens in the common cold.
Other viruses have a life history in which they sequentially infect different cell types. For example, the polio virus first infects the intestinal epithelium and then may infect cells in lymph nodes draining the intestine to which it travels in the lymph. In a small minority of cases, the virus also infects motor neurons in the spinal cord leading to muscular paralysis and thus preventing breathing; in the 1950s, this necessitated patients spending the rest of their lives in iron lungs to enable artificial respiration. Some, such as the influenza virus, are “one-hit” viruses – they cause an infection, but are rapidly cleared by the immune system. Others, once they have infected, may stay with us for long periods or a lifetime. Herpes simplex virus (HSV) infects epithelial cells in the oropharynx or genital tract, but then infects sensory neurons and travels to dorsal root ganglia in the spinal cord. Although you are unaware of it, once you have been infected, the virus will stay there for the rest of your life, often hidden from the immune system; this is called latency. If you have ever had a cold sore you will always have the virus. From time to time the virus becomes re-activated and travels back down the nerves to the skin, causing the typical lesions of cold sores. The same is true of chickenpox – once infected you always have the virus, but in this case if it is reactivated it causes the painful disease known as shingles.
To infect a cell, a virus has first to attach to the cell. It usually does this by binding to specific molecules on the cell surface. Thus, for influenza, haemagglutinin (HA; a molecule in the viral envelope) binds to sialic acid (a carbohydrate that is part of many glycoprotein molecules on the surface of cells). In the case of HIV, the envelope protein gp120 can bind to a molecule called CD4 on human T cells, as well as dendritic cells (DCs) and macrophages (which, in human, also express CD4). This binding specificity determines which cells a particular virus can infect; this is known as viral tropism. For example, polio virus can only infect human and some primate cells. However if polio virus RNA is injected into chicken cells, fully infectious virus can be formed because, once inside the cell, it can use the cellular machinery to replicate. The resultant viruses are able to infect human cells, but still cannot infect other chicken cells because they do not have the appropriate receptor. This shows that the tropism (host range) of this virus is determined by its ability to bind to molecules expressed by the host cell. Similarly, mice are normally totally resistant to polio. If, however, transgenic mice are constructed that express the human polio virus receptor, the mice can be infected and their cells release fully infectious virus. See Figure 2.3.
Figure 2.3 Life cycles of pathogenic viruses. Following transmission, viruses bind to specific molecules expressed by the host cell (this determines the cells that can be infected i.e. the tropism of the virus) and are then internalized by endocytosis or by direct fusion with a cell membrane. The viral nucleic acid is released and viral proteins are synthesized. Some proteins are involved in replication of the viral nucleic acid and others are used for assembly of new viral particles. Viruses can then be released from the cell either by budding from the membrane or by killing the cell.
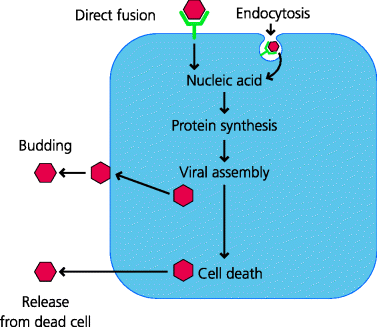
The attached virus may bind to the plasma membrane, after which its envelope fuses directly with the membrane, or may enter the cell by endocytosis. In endocytosis the cell membrane associated with the bound virus invaginates, and closes to form an endocytic vesicle containing the virus and some extracellular fluid. (In contrast, the uptake of a small particle such as a bacterium is called phagocytosis, and the vesicles formed are termed phagosomes.) Proteins in the virus enable the virus to fuse with the endosomal membrane and the viral nucleic acid can now enter the cytoplasm. For example, in influenza, the low pH in the endosome induces a conformational change in the viral HA, which permits it to fuse with the endosomal membrane. The viral nucleic acid hijacks the cell’s metabolic machinery and instructs the synthesis of viral proteins. In many cases, so-called early proteins are involved in the regulation of metabolism and the replication of the viral genome, followed by the synthesis of late proteins which are assembled to form the viral particles. The virus is released from the cells either by budding, in which case part of the infected cell membrane forms the viral envelope, or by causing the death of the cell, and allowing release of new viruses into the extracellular spaces from where they can infect new cells or new hosts.
2.2.2.2 Bacteria
Bacteria are small, self-contained organisms, generally capable of reproducing independently of host cells. They are present everywhere throughout our environment but almost all of them are harmless, and indeed some are very beneficial to their hosts. Bacteria are classified by their morphology and biochemical characteristics (e.g. cocci are round and bacilli are elongated). You may also see bacteria described as Gram-positive or Gram-negative. This refers to their staining properties with particular dyes and reflects the biochemistry of their cell walls. However, from the point of view of pathogenesis and immunity, it is most useful to classify bacteria in terms of the mechanisms by which they cause disease. This approach is used in the following sections. Unlike viruses, there is no generally applicable life history because bacteria have very diverse patterns of behaviour. Acquisition of bacterial infection is however by much the same mechanisms as for viruses, as shown by the following examples. They may be acquired from droplets in the air, as in bacteria that cause sore throats (Streptococcus pyogenes) or tuberculosis (Mycobacterium tuberculosis); from contaminated food and drinking water, as in bacteria that cause food poisoning (Salmonella) and diarrhoeal diseases (Vibrio cholerae; cholera); through sexual contact (Treponema pallidum; syphilis); or through cuts and abrasions (Clostridium tetani; tetanus) or insect bites (Yersinia pestis; plague). Often indeed they are derived from the hosts themselves: urinary tract infection originating from normal bacteria of the large intestine (Escherichia coli; cystitis) is an example we noted earlier.
Some bacteria do not need to infect or cross an epithelial surface to cause infection. Following inhalation, Streptococcus pneumoniae, the cause of lobar pneumonia, inhabits the smallest air spaces (alveoli) in the lung. After ingestion, Vibrio cholerae attaches to intestinal epithelium but does not invade. Some bacteria, however, do invade epithelia. Following ingestion, Shigella disenteriae (the cause of dysentery) invades intestinal epithelium but does not go further, while other organisms such as Salmonella typhi, the cause of typhoid fever, go even further. The latter first invades intestinal epithelium, but it then crosses the endothelium (the layer of cells that lines blood vessels, as well as lymphatic vessels) to enter the bloodstream and infect other sites such as the liver. Many bacteria that have crossed an epithelial surface nevertheless remain largely extracellular and for this reason they are termed extracellular bacteria. Others, however, particularly those mycobacteria that cause tuberculosis and leprosy, live preferentially inside host cells, often in fact primarily within macrophages, cells that normally play important roles in defence against this type of organism, The bacteria can therefore be termed intracellular bacteria. They, and some protozoal parasites such as Leishmania, can also be referred to as facultative intracellular parasites – the term parasite being used here in a generic rather than specific sense. See Figure 2.4.
Figure 2.4 Life cycles of pathogenic bacteria. Different types of bacteria cause very different types of diseases. Some pyogenic (pus-forming) bacteria such as Streptococcus can live and replicate extracellularly and may spread destructive infections to a variety of tissues. If not treated they can cause acute inflammation that resolves within a few days or can cause death, again in a few days. Other bacteria such as Mycobacterium tuberculosis which causes tuberculosis can only survive and replicate intracellularly. The immune response against the infected cells often causes chronic inflammation that typically lasts for months or years if not treated successfully.
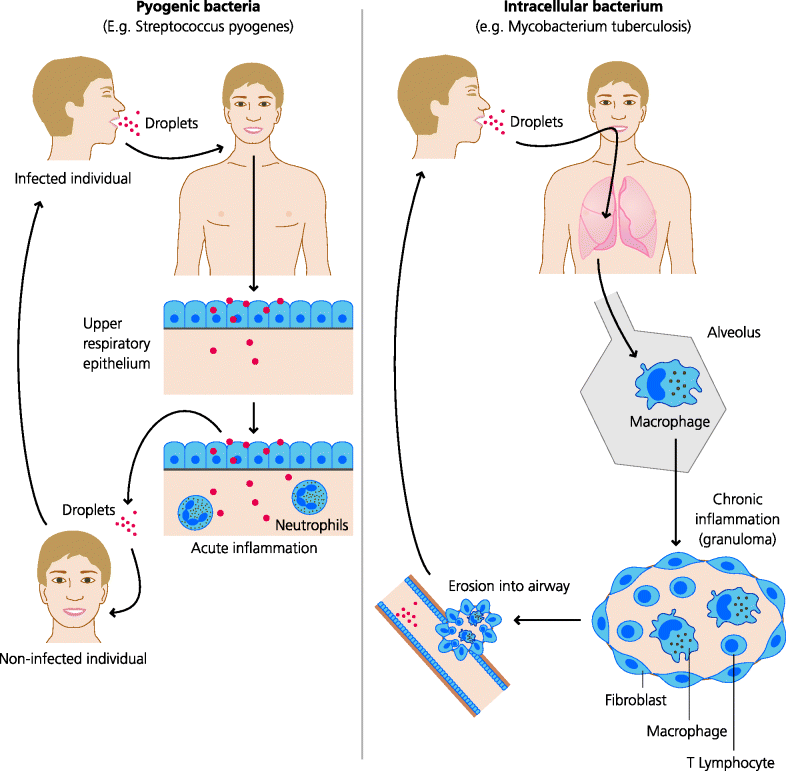
2.2.2.3 Fungi
Fungal infections are mainly acquired from the local environment. Their spores are everywhere and mostly they cause superficial infections: athlete’s foot, Dhobie itch (groin) and thrush (vulva and mouth) are common examples. Occasionally fungal infections may be widespread throughout the body (systemic), but this is rare in normal individuals although it can be a major problem in patients with immunodeficiencies such as AIDS.
2.2.2.4 Parasites
As noted above, a wide variety of different organisms are grouped together and classified as parasites, although the term is sometimes used more loosely to include certain bacteria as well (above). In fact all “true” parasites are, by definition, eukaryotes. The diversity of such parasites ranges from single-celled eukaryotic organisms such as protozoa, to multi-cellular metazoa such as worms. Parasitism refers to a symbiotic relationship in which the infectious organism lives on or in its host and obtains nourishment from it, often to the detriment of the host. Another form of symbiosis is commensalism, in which one partner benefits without apparently affecting the other. For example commensal bacteria may benefit the host by competing with other, potentially pathogenic bacteria. Nevertheless, it is clear that the commensal bacteria also benefit from this relationship (e.g. the host provides shelter and a source of nutrients) and as such this relationship actually reflects another form of symbiosis called mutualism in which both partners benefit. It is however difficult to see how true parasites could benefit their hosts in any way.
Parasites have hugely diverse life histories – sometimes undergoing remarkably different morphological (and sexual) changes at different stages – and it would be unwise to try to generalize as to how they are all acquired or how they all cause disease. Nevertheless some protozoal infections are transmitted to humans through insect bites. For example, the protozoa that cause malaria (Plasmodium) are transmitted by mosquitoes, those that cause African sleeping sickness and Chagas’ disease (Trypanosoma) by tsetse flies and assassin bugs, respectively, and Leishmania by sand flies. Others are acquired orally, such as the protozoa that cause amoebic dysentery (Entamoeba hystolitica) and toxoplasmosis (Toxoplasma gondii). After infection of their hosts, some protozoa live inside particular cells, such as Leishmania, which preferentially infects macrophages as noted earlier. Others can infect a variety of different cell types even at different stages of their life history. As we shall see (e.g. Section 2.4.5), the malarial parasite (Plasmodium falciparum) after infecting hepatocytes in the liver undergoes repeated cycles of infection of red blood cells. See Figure 2.5.
Figure 2.5 Generalized life cycles of parasites. Parasites display a huge variety of life cycles. In many cases there is an animal reservoir and a different animal vector. Often, as in schistosomiasis, the parasite exists in different forms in the reservoir, the vector and the human host. Schistosomes are metazoan parasites that live in water snails and are transmitted by their cerceriae forms that penetrate the skin of humans in the water. They migrate to the lungs, mature and adult sexual forms live in blood vessels in different sites. Some species live in blood vessels around the bladder and the eggs may then enter the bladder, and re-enter water via urine, to infect more snails.
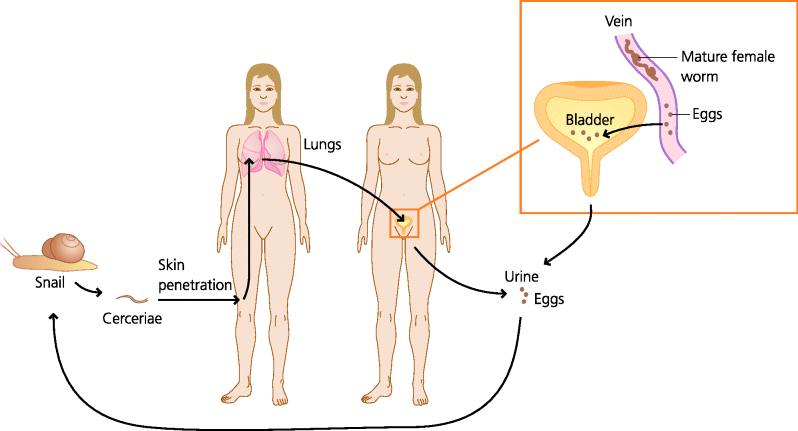
In terms of routes of transmission, similar principles to the above often also apply to worm infections. For example, the worms that cause filariasis (Onchocercidae) are transmitted by biting insects, whereas those that cause trichinosis (Trichinella spiralis) are acquired orally from food. Some have even evolved mechanisms to infect directly through the skin: after release by snails into the water, the parasites that cause schistosomiasis (Schistosome cercariae) can directly penetrate the exposed skin of humans that come into contact with it. In general, once they have infected, some parasites at different stages of their life cycle can invade a variety of different tissues, such as T. spiralis which infects the mucosae of the intestine, liver and skeletal muscles. Others, such as tapeworms, being so much larger, remain in the lumen of the intestine.
2.2.3 Infection and Disease
It is important to make a distinction between infection and disease. If a room full of immunocompetent individuals were subjected to an aerosol containing a pathogenic bacterium (e.g. Mycobacterium tuberculosis), everyone would inhale the bacterium, but only a small proportion, possibly around 10%, would develop clinical disease. The reminder would have been actively infected, but would have controlled the bacteria without any clinical sign of disease. In this latter case people were actually infected with the organism, in the sense that it gained access to their bodies, but with fully functioning immune systems they were able to recover without clinical signs of disease. Therefore, infection does not necessarily lead to disease. See Figure 2.6.
Figure 2.6 Clinical and subclinical infection. Most microbes that enter the body do not cause any symptoms: they are dealt with by the immune system silently so the infection is subclinical. Other microbes when they infect will almost always cause symptoms: the infection is clinically evident. Some organisms such as the fungus Pneumocystis jirovecii do not cause clinical infection in normal humans, but can do so if the immune system is defective, as in AIDS; these infections are opportunistic. Opportunistic infections tell us that in normal individuals the immune system is working continually to eliminate or control many microbes.
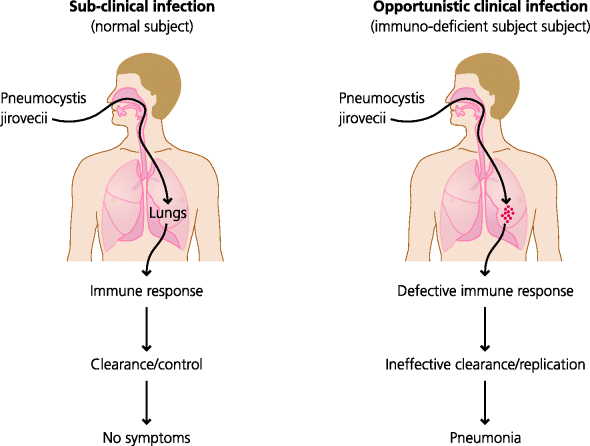
What properties does a microbe need to possess if it has the potential to cause disease in normal individuals?
It is not uncommon for an individual who has recovered clinically from an infection and is hence asymptomatic, or who has never had clinical symptoms, to be infectious to others. These individuals are known as carriers. The most famous example is Typhoid Mary – a cook in New York at the start of the twentieth century – who, after recovering from typhoid, retained live bacteria in her gall bladder from which they were excreted into her faeces. It is said that she managed to infect over 40 people, several of whom died, presumably because of inadequate personal hygiene. Many other examples of carrier states exist: hepatitis B, hepatitis C, HIV, methicillin-resistant Staphylococcus aureus (MRSA), syphilis, meningococcus (Neisseria meningitidis) and gonorrhoea (Neisseria gonorrhoeae) are some of the better known.
2.2.4 Immunodeficiency Diseases
Some individuals suffer from an unusual frequency or pattern of infectious disease, often becoming apparent in very early life. These conditions may represent an inability to mount an effective immune response against an infection and may be inherited or acquired. These are the immunodeficiency diseases. If a child is born with a mutation in a gene encoding a molecule important in immunity to infection, the defect may result in the child becoming infected with common pathogens more frequently than normal individuals. They may also become infected with microbes that do not give rise to clinical infections in individuals with normal immunity. Often these are evident as severe, persistent, unusual or recurrent (SPUR) infections. These genetic defects, which can be inherited in an autosomal or X-linked manner, are known as primary immunodeficiencies. They differ from infections that are secondary to another cause, such as in individuals with advanced cancer or who have been aggressively treated for cancer, or, as we have noted earlier individuals who have been infected with HIV and have progressed to AIDS. These latter conditions are called acquired or secondary immunodeficiencies.
Primary immunodeficiencies can result from defects in genes involved in innate immunity or, more frequently, in adaptive immunity. Defects in innate immunity include mutations in genes that encode proteins involved in the recruitment of phagocytes to inflammatory sites or in the ability of phagocytes to kill bacteria, e.g. leukocyte adhesion deficiency (LAD) and chronic granulomatous disease (CGD), respectively, as well as in structural or regulatory components of the complement system (complement deficiencies). Defects in adaptive immunity include mutations in genes that encode proteins which are essential for normal lymphocyte development, so that T cells and/or B cells are absent or defective, or which are needed for T cells to help B cells make different types of antibodies, e.g. severe combined immunodeficiency (SCID) and hyper-IgM (HIGM) syndrome, respectively.
Around 200 different primary immunodeficiencies are now recognized and the genetic bases of more than half of them have been identified to date. These immunodeficiencies are very rare, but are highly informative because they provide evidence for the role of those genes – and of the mechanisms they regulate – in normal immunity. We shall meet again some of those mentioned above, and others, later in this chapter, and we discuss some of them in more detail in Chapters 3–6, sometimes illustrating them with case studies.
Secondary (acquired) immunodeficiencies are similarly varied in their nature. Some, such as AIDS, are well understood, even if we can do little about them. Others, such as those accompanying infections such as measles, are much less well understood.
2.2.5 Exploring Immunity to Infection
Before we start discussing the ways in which we combat real infections, it is important to think about evidence: how is it that we can know which molecules, cells or tissues are important in defence against any particular pathogen? This type of evidence can be obtained from different settings.
First, as we outlined above (Section 2.2.4), we have studies of human disease. The roles of different defence mechanisms in combating infection are often most clearly demonstrated by individuals who for one reason or another cannot generate a particular mechanism. We can then ask, what kind of infections do they acquire? This in turn provides evidence for the normal role of that mechanism in host defence against the particular type of infectious agent. Thus, the value of studies of these patients in understanding immunity to infection cannot be over-emphasized. In some cases, however, the deficiency may have been identified in only one or two extremely rare individuals, so that care is still needed in extrapolating some of these observations to the whole population.
Second, we have studies in experimental animals. Animal experiments are crucial in that they allow the dissection and analysis of immune responses in ways that can never be achieved in humans. A good example of this comes from the use of genetically modified mice (e.g. mice in which a gene has been “knocked out” so that its product can no longer be expressed). Thus if a patient with increased susceptibility to a particular kind of infection is found to have a defect in the production of a protein, say a receptor for a cytokine such as the interferon (IFN)-γ receptor, this defect is a good candidate for the molecular basis of the increased susceptibility, but of course there may be other defects that have not been identified. If, however, the gene coding for that protein is inactivated in a mouse (and only that gene is inactivated) and the mouse shows increased susceptibility to a similar infection, this is much stronger evidence that the candidate gene is in fact crucial in the development of immunity to that kind of infection. A word of caution, however: mechanisms of immunity that have evolved in humans are sometimes different from those that are found in mice, so sometimes gene knock-outs in mice do not fully recapitulate the human diseases. See Figure 2.7.
Figure 2.7 Gene-targeted, knock-out mice. Embryonic stem (ES) cells from the blastocyst (an early stage of embryogenesis) have the potential to develop into any cell or tissue of the body. Techniques have been developed to maintain these cells indefinitely in culture. DNA containing a gene whose sequence has been disrupted can then be inserted (transfected) into ES cells. In tissue culture the stem cells divide and, on rare occasions, the inserted gene will replace the normal gene through the process of homologous recombination. The altered ES cell can then be injected into an early mouse embryo, inserted into a “pseudo-pregnant” female, and may enter the germline. One copy of the defective gene may then be expressed in the progeny of the resulting mouse. By interbreeding mice expressing one copy of the defective gene, mice homozygous for the defective gene may be selected. These mice will not express the protein encoded by the normal gene because that gene has now been “knocked out”.
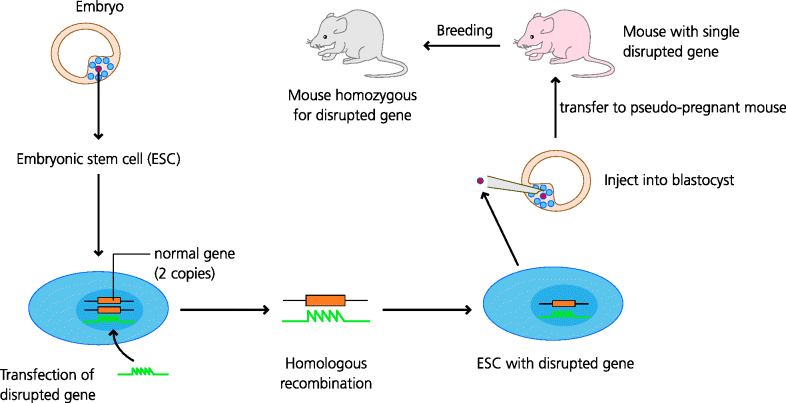
Another, third, approach is more active. Experimentally, resistance to infection can often be transferred to a non-immune animal by transfer of the appropriate immune effector mechanism. This is known as adoptive transfer – a most important approach in immunological research. Thus, cells such lymphocytes or lymphocyte subpopulations, or serum containing antibodies, can be transferred from an immune animal to a normal animal and the recipient tested for immunity. Although this approach is most often used experimentally, in some instances adoptive transfer is used to treat or prevent human disease; for example, antibodies raised in horses or humans have been used to treat tetanus, diphtheria and hepatitis. Hence, the effectiveness of these latter treatments is very strong evidence for the potential of antibodies to mediate defence against these the causative microbes. See Figure 2.8.
Figure 2.8 The principle of adoptive transfer of immunity. If an animal is vaccinated against a pathogenic microbe (or has recovered from an infection) it is usually resistant to re-infection with the same microbe. To find out which part of the immune system is mediating this resistance, different immune components such as antibodies in serum or T cells isolated from lymphoid tissues can be transferred to a normal animal; this procedure is known as adoptive transfer. The recipient can then tested for resistance to the microbe. In the case shown, T cells, but not antibodies, are needed for defence against this particular pathogen.
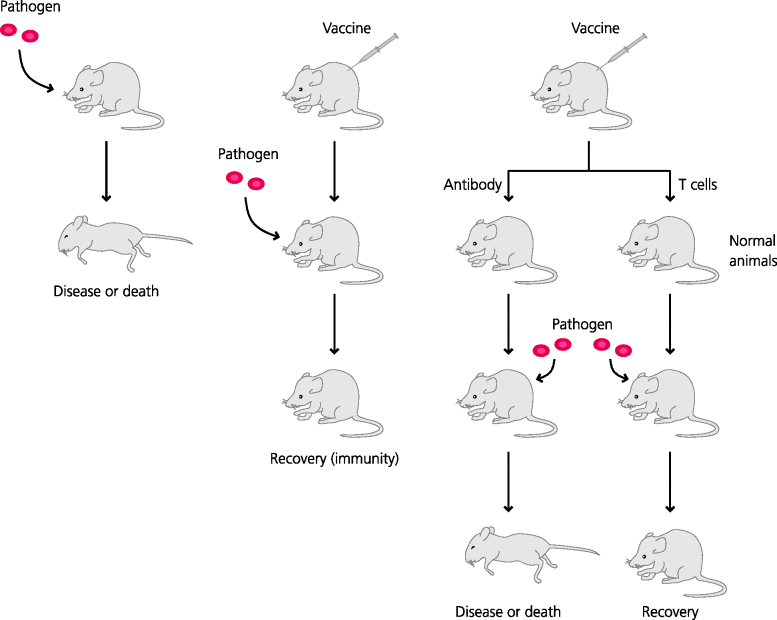
2.3 Host Defence Against Infection
In this section we will give an overview of the different mechanisms that are used to defend against infectious disease. To illustrate how each component of the immune system can contribute to host defence, we highlight specific types of infectious agent that can be eliminated by each. This approach is of course an over-simplification, as multiple mechanisms are usually involved in immune responses against any given potential pathogen, but it enables us to establish general principles, after which the exceptions can be better understood. Then, in Section 2.4, we bring these mechanisms together, and emphasize the ways in which innate and adaptive mechanisms can be integrated to provide effective protection. One important principle that should be stressed is that because the different types of pathogen vary so much in size and life history, very different mechanisms are needed to deal with them. Another is that because hosts and pathogens have co-evolved they have generally reached a “balanced” relationship despite disease. See Box 2.1.
2.3.1 Mechanisms of Innate Immunity
2.3.1.1 Phagocytes and Phagocytosis
Phagocytosis is the internalization of particles by cells. Macrophages, and neutrophils, polymorphonuclear neutrophils (PMNs), are specialized phagocytes and are the most important phagocytes in defence against infection. They are able to phagocytose small micro-organisms such as bacteria, small parasites (protozoa) and some fungi, and they also internalize viruses. This is an important mechanism leading to the intracellular elimination of some types of infectious agent. Its importance is shown by patients in whom phagocytosis is defective or who cannot recruit phagocytes to inflammatory sites and who consequently suffer from an increased incidence of infection; LAD (see Chapter 4) is an example of the latter type of defect. See Figure 2.9.
Figure 2.9 Endocytosis and phagocytosis. All cells can sample their extracellular milieu through the process of endocytosis. All can take up molecules in the fluid-phase by pinocytosis or through receptor-mediated endocytosis, into endosomes. Specialized cells such as neutrophils and macrophages can also internalize particles by phagocytosis. During phagocytosis, sequential interactions of cell surface receptors and ligands on the particle may result in a zippering process involving the actin cytoskeleton that leads to the particle being enclosed within the cell in a phagosome. Some cells (especially some DCs) can extend large sheets of cytoplasm that fuse to enclose large volumes of fluid; this is macropinocytosis. The internalized vesicles may then fuse with lysosomes that contain degradative enzymes. As the endolysosomes mature they become increasingly acidified, resulting in activation of the degradative enzymes (e.g. acid proteases) that degrade their contents. Sometimes internalized receptors are recycled back to the surface to be re-utilized (not shown).
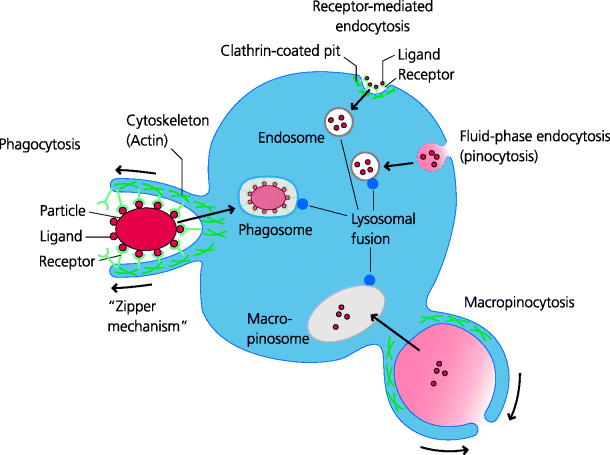
In phagocytosis, the micro-organism is recognized by cell surface receptors, which often include certain pattern recognition receptors (PRRs) (Section 1.2.3.1). Subsequently it is internalized into a membrane-bound vacuole called a phagosome. The phagosome then fuses with cytoplasmic organelles, called lysosomes, which contain pre-formed anti-microbial toxins and digestive enzymes. Often the micro-organism can be killed and degraded in this way. Neutrophils and, to a lesser but no less important extent, macrophages use phagocytosis as a crucial mechanism of host defence. This ensures that many infectious agents are not pathogenic to normal individuals because they can be so rapidly phagocytosed and eliminated early after infection. It should be emphasized that phagocytosis by macrophages is also an essential part of normal homeostasis, such as in the removal of aged erythrocytes and the elimination of dying (apoptotic) cells during tissue remodelling.
2.3.1.2 Opsonization, Complement and Natural Antibodies
Phagocytosis is an essential first stage in the killing of many viruses and other microbes. Because they are so small, many viruses are actually internalized through receptor-mediated endocytosis. Not surprisingly, many pathogens have evolved means of avoiding phagocytosis (e.g. by changing the structures of their external surfaces). In turn, vertebrate hosts have evolved additional mechanisms that are nevertheless still able to direct such pathogens into the phagocytic pathway. This is the process of opsonization. For example, microbes can be coated by specialized host molecules such as complement components or, usually later after infection, with antibodies. These can subsequently be recognized by cell surface receptors on phagocytes to stimulate phagocytosis. See Figure 2.10.
Figure 2.10 Opsonization. Opsonization prepares a particle for phagocytosis by coating it with molecules for which phagocytes have receptors that can mediate internalization. For example, some bacteria can be phagocytosed directly via PRRs (not shown) and are often non-pathogenic. Pathogenic bacteria may have developed capsules to protect themselves from uptake in this way, but can be taken up after they have been opsonized. The main opsonins are antibodies and certain complement components that are bound directly to the surface of microbes, or to antibodies that are attached to them (mainly IgM and some classes of IgG). A variety of different Fc and complement receptors can mediate internalization of antibody- and complement-opsonized particles, respectively.
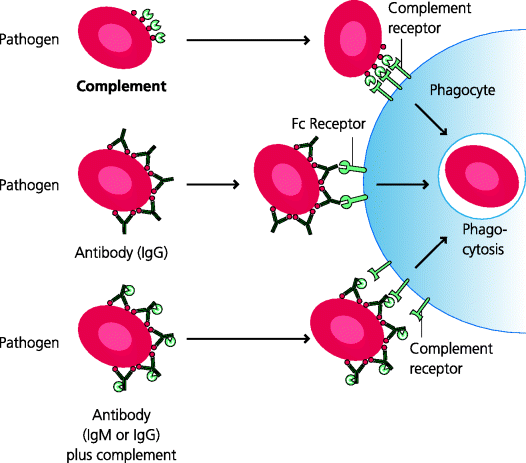
The complement system consists of a large number of soluble molecules that circulate in the blood. Together they constitute a proteolytic cascade in which initial activation, by three different routes, results in amplification of the numbers of activated molecules generated at each stage. The deposition of certain activated complement components on a microbe enables them to act as opsonins: their subsequent recognition by complement receptors enables phagocytosis of the opsonized microbe. Other complement components are involved in the induction of acute inflammation. The components activated later in the complement pathway may also assemble into pores (the membrane attack complex) on bacterial surfaces leading to lysis of some types of bacteria. The complement system appears to be particularly important in defence against extracellular, pyogenic bacteria because individuals with genetic defects of the so-called central and late components which are common to all three pathways show an increased incidence of such infections. Pyogenic means pus-forming, and the pyogenic bacteria are typically those such as Staphylococcus aureus and Streptococcus pyogenes that cause acute inflammation and the formation of pus at superficial sites. See Figure 2.11.
Figure 2.11 Complement activation and production of opsonins. Complement comprises a large group of proteins, some of which can be activated sequentially in a cascade. Three main pathways are involved in activation. The classical pathway typically starts with C1q binding to antibodies on the surface of a particle. The lectin pathway often involves mannose-binding lectin (MBL) binding to carbohydrates on a particle. The alternative pathway utilizes the continual activation of C3 on particle surfaces and serves to amplify the other pathways. These pathways all come together with the activation of component C3. Its products C3b, and particularly iC3b, act as potent opsonins through binding to complement receptors such as CR3 and CR1.
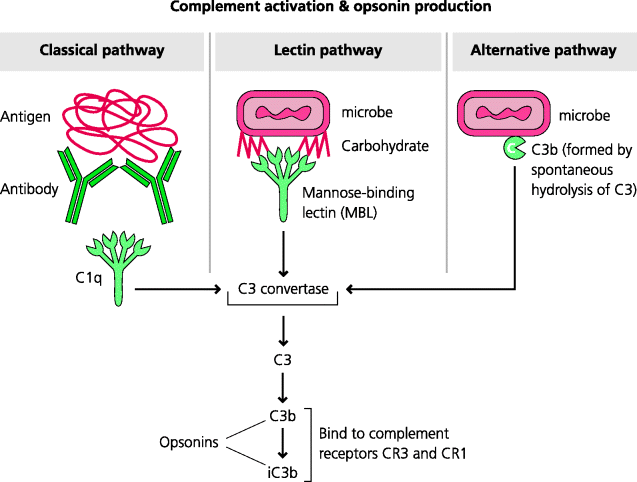
Antibodies can also act as opsonins on their own, or after activating complement and subsequently leading to uptake via complement receptors (as above). Even before adaptive responses are mounted, so-called natural antibodies are produced that have weak affinity for a variety of infectious agents and which can generally activate complement. The deposition of other types of antibodies on a micro-organism can enable its subsequent recognition by another set of phagocyte receptors, termed Fc receptors (FcRs), some of which induce phagocytosis.
2.3.1.3 Neutrophils, Extracellular Bacteria and Fungi
Neutrophils are the most common leukocytes in blood, and hence they can be rapidly recruited to sites of infection and inflammation. Substantially more neutrophils can be mobilized from the bone marrow as the infection progresses. Extracellular pyogenic bacteria, which can multiply rapidly outside host cells, require a fast host response. Owing to their large numbers and rapid mobilization, neutrophils play an essential role in the elimination of such bacteria as well as some types of fungi. Neutrophils have several mechanisms for killing bacteria. Particularly important is the intracellular production of reactive oxygen intermediates (ROIs), including hydrogen peroxide (good if you want to bleach your hair) and hypochlorous acid (the main component of domestic bleach). These ROIs are secreted into the phagocytic vacuole and can be highly toxic to many bacteria. Lysosomal proteases, which are secreted into the vacuole, are important in digesting bacteria but may also have a direct role in killing. In neutrophils, ROIs may facilitate this killing by actually helping to increase the acidity of the vacuole. In addition, neutrophils extrude chromatin and certain enzymes that may trap and digest bacteria extracellularly in more-recently identified structures termed neutrophil extracellular traps (NETs). Having done their job, the neutrophils die. Other mediators they produce help to digest and liquefy the local tissue (in preparation for repair and reconstruction) and this liquid, together with dead and dying neutrophils, forms pus. See Figure 2.12.
Figure 2.12 Neutrophil microbicidal mechanisms. Phagocytosed bacteria are subjected to a variety of potential killing mechanisms within the phagosome. These include reactive oxygen intermediates, anti-microbial proteins and peptides, and lysosomal enzymes. Neutrophils may also be able to secrete or release anti-microbial mechanisms that act extracellularly. These include anti-microbial proteins and peptides such as defensins, and NETS consisting of extruded sheets of nuclear material that may serve to trap bacteria, facilitating their killing.
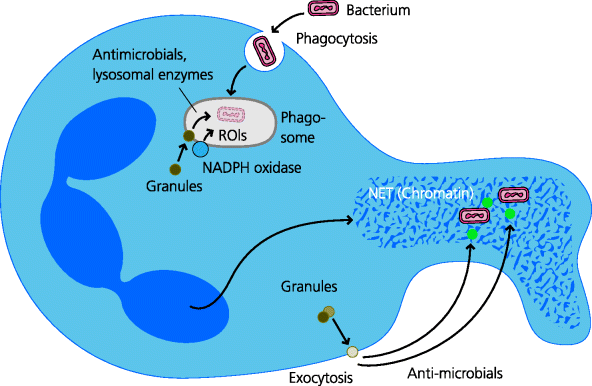
We know neutrophils are important in particular types of infection because patients with reduced numbers of neutrophils or genetic defects, such as the inability to generate ROIs, become highly susceptible to infection with some extracellular pyogenic bacteria and fungi; CGD (see Chapter 4) is an example of this type of defect. Some of the infectious agents that more readily cause disease in these patients are not pathogenic in immunocompetent individuals. (That they do infect people with defective neutrophils tells us that, in immunocompetent people, neutrophils are working away all the time without us being aware of it.) In particular, these patients may have an increased incidence of infection with pyogenic bacteria such as Staphylococcus aureus. It is important to realize that patients with neutrophil defects do not show an increased susceptibility to other infectious agents such as intracellular bacteria (e.g. mycobacteria) and viruses. This is good evidence that neutrophils do not normally play major roles in defence against these types of organism.
2.3.1.4 Macrophages, Intracellular Bacteria and Viruses
In contrast to neutrophils, macrophages are normally resident within almost every tissue of the host. Microbial recognition by PRRs on tissue-resident macrophage is an important stimulus in the initiation of inflammation because they stimulate secretion of cytokines and chemokines. When infection causes inflammation, monocytes are recruited to the site of infection and develop into macrophages or, under influences that are still unclear, into dendritic cells (DCs).
Unlike neutrophils, normal tissue-resident macrophages have limited anti-microbial defences, probably because they are usually pre-occupied with other homeostatic duties (tissue remodelling and such-like). Potent anti-microbial activities, including the production of ROIs, can, however, be induced in macrophages (perhaps mainly in the newly recruited macrophages) by cytokines produced by other cells, particularly IFN-γ. This cytokine may be produced, at early stages of infection, by natural killer (NK) cells and, later, by a subset of activated (Th1) T helper cells. The importance of such signals is highlighted by individuals who, for example, have genetic defects in the IFN-γ receptor and who become particularly susceptible to infection by intracellular bacteria. Many of these bacteria are considered to be commensals in normal individuals, again showing the importance of subclinical activity of the immune system.
Phagocytosis by macrophages can, however, be a double-edged sword. If a pathogen can survive inside a macrophage, the longevity of these cells can provide a safe haven. Such pathogens have evolved a variety of mechanisms to permit their survival inside macrophages. For example, Listeria can escape from the phagosome into the cytosol, avoiding the killing mechanisms which are directed into the phagolysosome, and pathogenic Mycobacterium prevent the fusion of phagosomes with lysosomes. In addition to this, the activated macrophage is a rather nasty customer – it is actively secretory, releasing molecules such as hydrogen peroxide which are toxic to many neighbouring cells, and proteolytic enzymes such as collagenase and elastase that break down connective tissue. Thus activated macrophages, as well as being the only cells that can kill pathogens such as Mycobacterium tuberculosis, are also the cells responsible for the tissue destruction that is so typical of chronic inflammation, as we shall see later (Section 2.4.2.3). See Figure 2.13.
Figure 2.13 Microbial macrophage evasion mechanisms. Many pathogens have evolved mechanisms to help them avoid being killed by macrophages. Some general mechanisms used by three different types of bacteria are shown for Listeria, Mycobacterium tuberculosis and Legionella (the causative agents of diseases such as listeriosis, tuberculosis and Legionnaire’s disease respectively); the relevance of the coiling mechanism of phagocytosis that is induced by the latter is not known. Many other evasion mechanisms have been discovered; for example, some bacteria may interfere with signalling pathways such as that stimulated by IFN-γ, thus inhibiting macrophage activation (not shown). Many others are probably yet to be discovered.
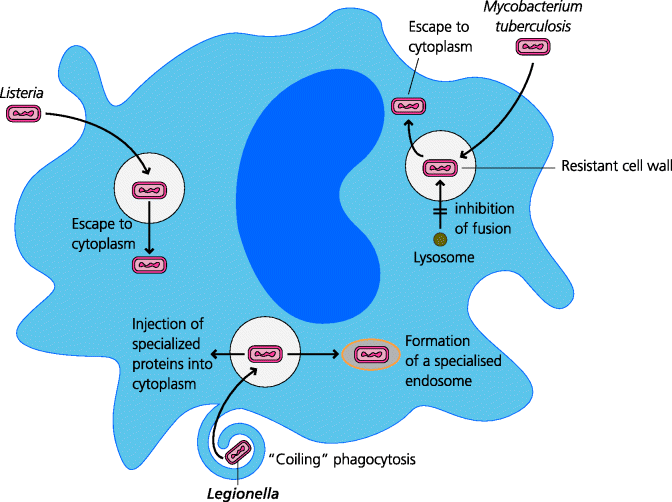
2.3.1.5 Natural Killer (NK) Cells and Viruses
Another cell involved in innate immunity is the natural killer (NK) cell. Some NK cells are present in the blood and blood-filtering organs such as the spleen, liver, lungs and bone marrow, and others can also be recruited to inflammatory sites. They can contribute to early responses against some bacteria because they can be triggered to secrete cytokines. For example, the cytokine IL-12 produced by other cells, such as macrophages or DCs, can trigger these cells to secrete IFN-γ. Another important feature of NK cells is that they can directly kill other infected host cells; unlike cytotoxic T cells, which need to be activated before they become cytotoxic (Section 2.3.2.2), NK cells show spontaneous cytotoxicity.
Viruses, once intracellular, can evade many host defence mechanisms including killing of infected cells by cytotoxic T cells. In some cases, however NK can recognize these virally infected cells and kill them. If the NK cell kills an infected cell before new virus has been released, it can limit viral replication and spread. Killing of virally infected cells by NK cells involves both the delivery of pre-formed granule contents or interaction with death-inducing receptors, leading to apoptosis of the target cell. Clinical and experimental evidence suggests that NK cells play particularly important roles in host defence against some viruses. This is demonstrated by very rare individuals with reduced numbers of NK cells or whose NK cells are defective at killing. Such patients suffer very severe, potentially fatal, infections with herpes viruses, but, if they can survive until the adaptive immune response kicks in, they may survive as well as normal individuals. See Figure 2.14.
Figure 2.14 Some functions of natural killer cells. NK cells are developmentally related to lymphocytes but are considered as belonging to the innate arm of immunity. Two main types of function are highlighted. Immune regulation. NK cells can regulate the functions of other cell types. For example, a feedback loop triggered by IL-12 secretion from macrophages can stimulate IFN-γ production by NK cells which in turns helps macrophage activation. Cellular cytotoxicity. NK cells can kill other cells. Different recognition systems enable NK cells to deliver pre-formed granule contents or to ligate “death-inducing” receptors and induce apoptosis (e.g. in cells infected with certain types of virus).
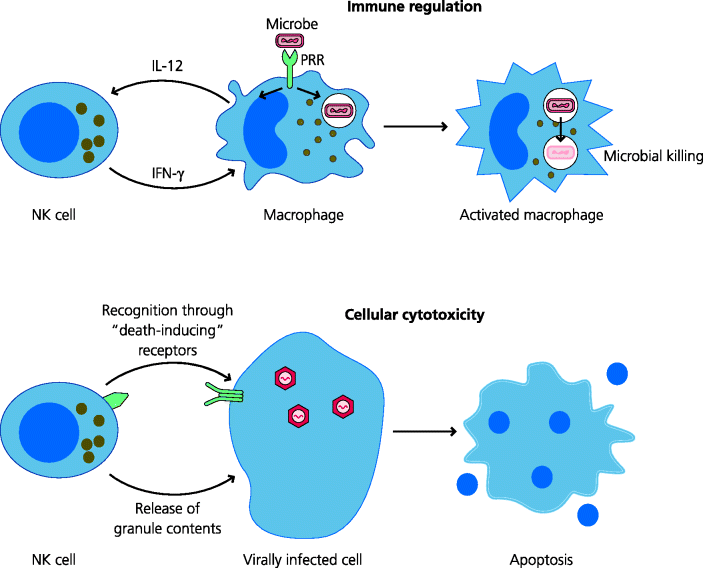
2.3.1.6 Mast Cells and Basophils, Extracellular Bacteria and Parasites
Mast cells are present in all loose connective tissues. They express PRRs and can rapidly produce pro-inflammatory cytokines that trigger inflammatory responses when they sense infection (i.e. these cytokines can be viewed as being alarm signals that are produced in response to the danger posed by infectious agents). Mice that lack mast cells may be particularly susceptible to infection by extracellular bacteria because mast cells are needed to recruit neutrophils (Section 2.3.1.3) which probably deal best with this type of pathogen. Mast cells also store pre-formed mediators of inflammation such as histamine, and can later synthesize lipid metabolites such as prostaglandins and leukotrienes which are important in mediating the vascular changes in inflammation (venular vasodilatation and increased permeability). See Figure 2.15.
Figure 2.15 Mast cell activation, degranulation and secretion. Mast cells reside in connective tissues and can be activated in several ways. They express receptors for complement components C3a and C5a, and a selection of PRRs such as Toll-like receptors (TLRs) that recognize microbial pathogen-associated molecular patterns (PAMPs). They can also be activated by cross-linking pre-formed IgE bound to FcRs on their surface. Activation results in very rapid degranulation with the release of pre-formed granule components such as histamine and cytokines. Mast cells can then synthesize and secrete other pro-inflammatory molecules such as lipid mediators (leukotrienes, prostaglandins) and more cytokines. Mechanical damage to mast cells can also lead to the release of histamine.
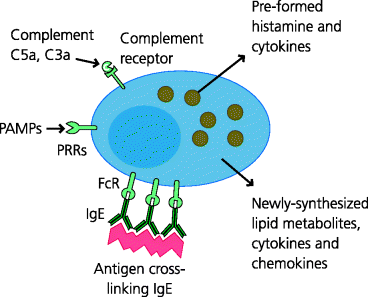
Mast cells are also present in mucosal tissues. Here they may play an important part in immunity to parasites, especially worms. Some of the lipid-derived mediators they produce increase the production of mucus, thus potentially adding a more effective barrier to access of worms to the gut wall. They may also cause smooth muscle contraction which might help to expel the worms in the faeces. Certainly mice lacking mast cells cannot clear some intestinal worms as effectively as normal mice under experimental conditions. However, given that we generally do not manage to get rid of most worms, their efficacy may be called into doubt.
A rare leukocyte, the basophil, somewhat similar to the mast cell, can be recruited from the blood to sites of some types of inflammation and infection. We still know relatively little about what these cells do. However, because basophils share many features with mast cells, they may be recruited to help to amplify the local responses that are typically stimulated by mast cells. Some more recent research also suggests they may play a role in polarizing CD4 T cell responses towards the Th2; type that typically occurs during parasitic infections (Section 2.3.2.1).
2.3.1.7 Eosinophils, Parasites and Tissue Repair
Eosinophils are normally rare in most connective tissues but are commonly found in mucosal tissues. Details of their roles in immunity to infection remain unclear. They have cytoplasmic granules that contain mediators that are known to be toxic to larger parasites, at least in vitro. Some of the functions of eosinophils overlap with those of mast cells (above). Both cell types, and additionally basophils, appear to respond to blood sucking ticks by degranulation at skin sites where ticks are feeding, although whether or how they might play a defensive role is not entirely clear.
Eosinophils (and indeed mast cells) additionally store or synthesize molecules that are important for tissue remodelling (e.g. growth factors for fibroblasts) and are present in tissues undergoing repair. Indeed, the Th2 types of adaptive immune response in which eosinophils (and basophils) are recruited may assist in the resolution of inflammation (production of anti- rather than pro-inflammatory cytokines), tissue repair and wound healing. In some cases, however, the excessive laying down of collagen can lead to loss of function in tissues, as can happen in chronic asthma. See Figure 2.16.
Figure 2.16 Eosinophil properties and functions. The functions of eosinophils are not fully understood. They are recruited into inflammatory sites such as those associated with parasitic infections and asthma. They express TLRs, and respond to cytokines such as IL-4, the chemokine eotaxin and some leukotrienes, for example. When activated they can secrete several cytokines including IL-4 and IL-13, and major basic protein which may be involved in defence against parasites. They can also mediate antibody-dependent cell-mediated cytotoxicity (ADCC) against some IgE- or IgA-coated parasites.
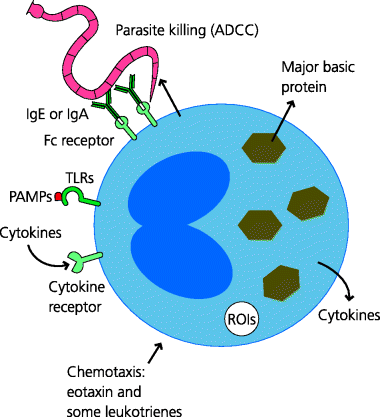
2.3.2 Mechanisms of Adaptive Immunity
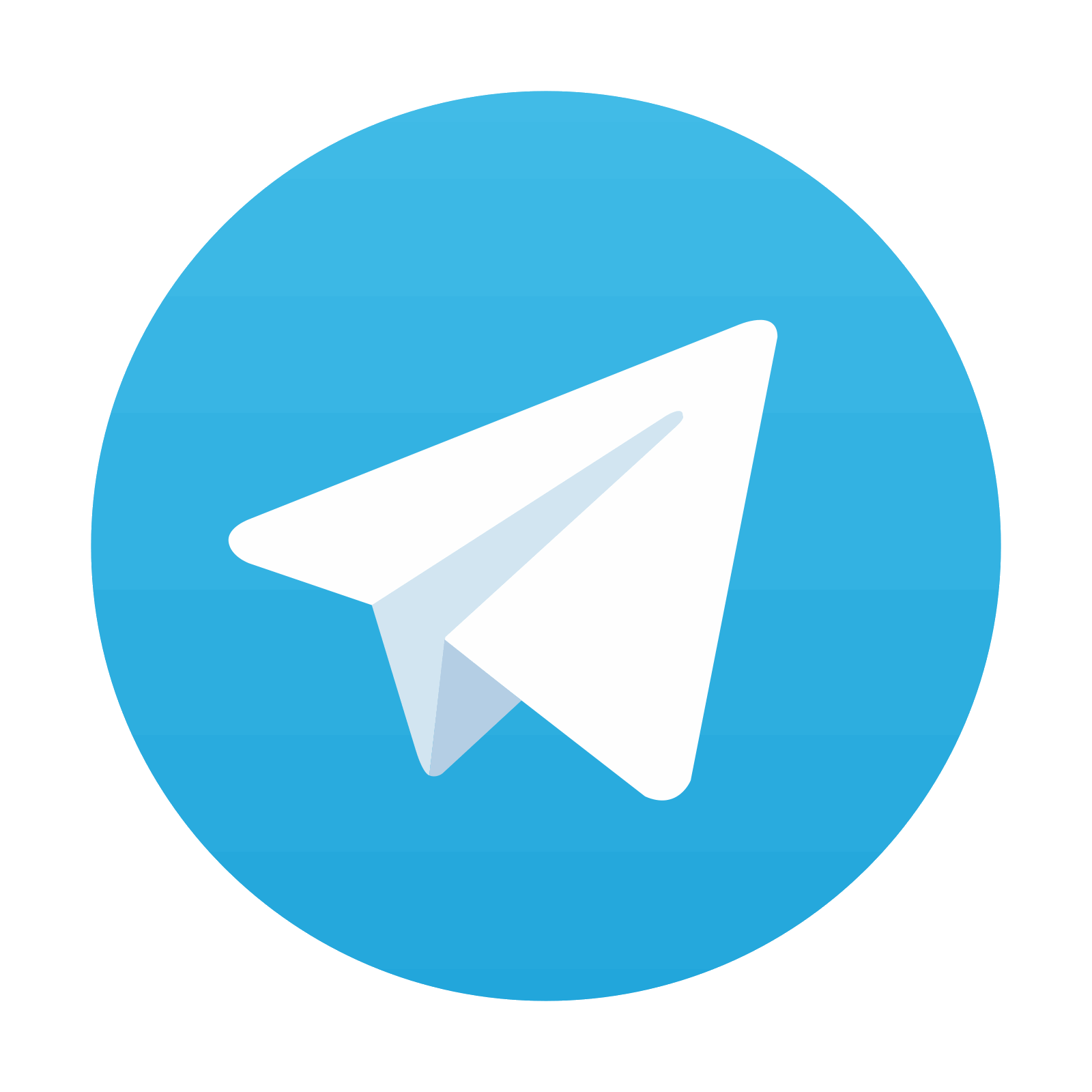
Stay updated, free articles. Join our Telegram channel
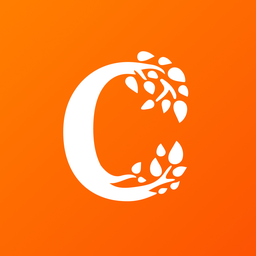
Full access? Get Clinical Tree
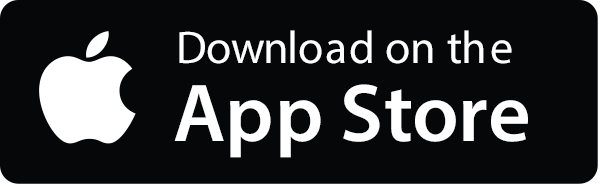
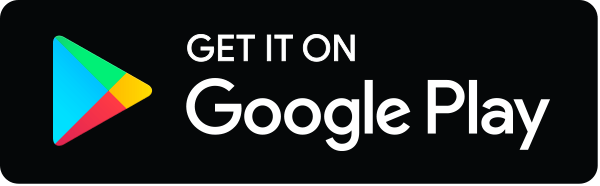