Chapter Outline
FUNCTIONAL GENETICS OF CHILDHOOD CANCER PREDISPOSITION SYNDROMES
DYSREGULATION OF CELL DIVISION AND GROWTH
DNA REPAIR AND GENOME INSTABILITY
SOMATICALLY ACQUIRED GENE MUTATIONS AND CANCER GENOMICS
GENOMIC REARRANGEMENTS AND CHROMOTHRIPSIS
DNA METHYLATION, CHROMATIN, AND THE EPIGENETICS OF CHILDHOOD CANCER
The first scientific reports of childhood cancers such as Virchow glioma (neuroblastoma), Wilms tumor (nephroblastoma), and Ewing sarcoma provided a classification system based on organ involvement but shed little light on the natural causes of these diseases. Subsequent study of cancer cells and animal tumors, and in particular Boveri’s analysis of cell mitoses and Rous’ study of oncogenic viruses, revealed that cancer is at least in part a disease of genes and their aberrations.
With the advent of molecular biology, we learned that animal oncogenic viruses contain mutant versions of cellular genes, termed oncogenes, as well as gene products that negatively regulate endogenous tumor suppressor genes. Expressed in normal cells, these tumorigenic viral genes can cause unrestrained proliferation and, ultimately, immortalization and malignant transformation, leading to the concept that human cancer is caused by the dysregulation of endogenous protooncogenes and tumor suppressor genes.
In particular, the idea of endogenous tumor suppressor gene inactivation in human cancers is strongly supported by (1) the analysis of the age dependence of retinoblastoma incidence and (2) the finding that in children who inherit one mutant allele, bilateral tumors develop at an earlier age than in persons who have tumors that develop as a result of spontaneous biallelic inactivation. Although this “two-hit” model provides a powerful explanation of the incidence of retinoblastomas, similar analyses of other human cancers led to the conclusion that at least six different genetic mutations are needed to explain the apparent age-dependent incidence of some human tumors. Indeed, a large majority of human cancers contain many gene mutations in a complex clonal architecture, as revealed by recent analysis of tumor genomes by next-generation sequencing. Some tumors, however—particularly those in children—can have surprisingly low mutation rates. Finally, even cancers with extremely low numbers of gene mutations contain mutations outside of genes that could contribute to tumorigenesis. Consequently, the extent to which alterations in noncoding genetic elements and epigenetic changes have the potential to cooperate with or substitute for genetic mutations has become an active area of investigation.
In addition to the polygenic nature of human cancers, many features of tumor cells are not directly explained by the mutation of cancer-causing genes but instead involve complex cell and tissue biologic systems. These “hallmarks of cancer,” which include limitless cell replication, self-sufficiency in growth signaling, circumvention of cell death, immune system evasion, and the ability to metastasize, provide an expedient phenomenologic model to understand cancer cell behavior.
Recently, contributions from changes in the chemical modifications of deoxyribonucleic acid (DNA) and chromatin have been increasingly implicated in cancer pathogenesis. These changes are often referred to as “epigenetic” because they may have the potential to result in somatically heritable changes in gene expression and phenotype that do not result directly from changes in DNA composition. Normal development provides a vivid illustration of the power of epigenetic changes, because virtually every cell in the body contains mostly identical coding DNA sequences and yet cells display markedly different behaviors and fates. For example, mature neurons have a distinct differentiated appearance and never divide, whereas hematopoietic stem cells divide throughout a person’s lifetime. Further, these cellular fates remain stable over years of somatic cell division. Given the role of epigenetic regulation in the control of cell fate, perhaps it is not surprising that epigenetic perturbations may also contribute to oncogenesis. Although they are conceptually distinct, genetic and epigenetic dysregulation can be closely associated in cancer cells, while genetic mutations of epigenetic regulators are increasingly recognized, particularly in childhood tumors.
Whereas somatic cancer mutations are relatively easy to identify by comparing the sequence of DNA between tumor and nontumor cells, epigenetic causes of cancer are much more difficult to discern because the normal epigenetic state varies with cell type, which makes defining aberrant features a challenge. With respect to DNA methylation, it has become evident that cancer genomes overall tend to be hypomethylated compared with normal tissues and that they have focal areas of hypermethylation in the promoters of specific genes associated with silencing of gene expression. Similarly, the recent discovery of mutations in genes that regulate chromatin structure suggests that aberrant chromatin modifications may contribute to tumorigenesis via epigenetic dysregulation rather than through the induction of additional genetic mutations, although in most cases this conjecture has yet to be proven.
Arguably, our ability to decipher the biologic basis of human cancer will depend on understanding the relationship between molecular aberrations and dysregulated properties of cancer cells. Awareness of the importance of this problem is increasing in the field of pediatric oncology, leading to the recognition that although some childhood tumors can have a remarkably low number of recurrently mutated genes, others can contain thousands of DNA substitutions and complex chromosomal rearrangements. Pediatric cancers may be conceptually distinct from those that occur in adults, because in the absence of a syndromic predisposition, children otherwise have relatively low rates of somatic genetic alterations and toxin exposures due to aging. Thus childhood cancers may involve unique mechanisms of tumorigenesis.
In this chapter we review the mechanisms of molecular pathogenesis of childhood cancers. Our goal is not to provide a comprehensive encyclopedia (electronic publishing and online databases * now enable ready access to this information) but to develop a systematic classification of the molecular lesions responsible for childhood tumors, with a focus on biologic mechanisms and functional principles. Instead of organizing this chapter on the basis of cancer types, which are covered individually in other chapters of this volume, we have attempted to develop a functional framework that we hope will provide helpful insights into the relationship between molecular aberrations and behaviors of cancer cells sui generis . Such an approach should inform the development of improved clinical therapies, particularly if cancer treatment is to become more precise through the use of rationally targeted agents.
Functional Genetics of Childhood Cancer Predisposition Syndromes
* At the time of this writing, the following publicly accessible databases of cancer-associated mutations and structural aberrations are available: http://target.cancer.gov ; http://pediatriccancergenomeproject.org ; http://www.sanger.ac.uk/genetics/CGP ; and http://cansar.icr.ac.uk .
The study of childhood cancer predisposition syndromes is an important source of information about the genetic basis of human cancers. Indeed, the retinoblastoma 1 (RB1) gene, which is mutated in familial retinoblastomas, was the first human tumor suppressor gene identified. What follows is an overview of known genes mutated in childhood cancer predisposition syndromes based on the major cell biologic pathways thought to be perturbed by the inherited gene mutations.Dysregulation of Cell Division and Growth
A large number of childhood cancer predisposition syndromes involve mutations of genes that regulate cell growth and division. One of the first to be described, the RB1 gene, is mutated in children who are predisposed to the development of retinoblastoma, pinealoblastoma, and osteosarcoma. The RB1 gene is part of the G1 cell cycle checkpoint, where its product directly binds and regulates the activity of the E2F family of transcription factors and the DREAM chromatin remodeling complex in response to stress. Cancer-causing deletions and nonsense mutations of RB1 can cause entry into the cell cycle by otherwise quiescent cells and mitigation of senescence that limits cellular life span. Whereas loss of RB1 in cellular and mouse models causes defects in mitotic chromosome segregation that lead to aneuploidy, most human retinoblastomas are largely diploid, suggesting that the canonic chromosome segregation effects of RB1 inactivation in human retinoblasts are functionally compensated. Human retinoblastomas invariably exhibit biallelic inactivation of RB1 , but otherwise often have a comparatively low mutation rate. One study found that RB1 was the only known cancer gene mutated in these tumors. However, additional subclonal DNA mutations were observed, with some mutations located outside of coding regions, which may cooperate with RB1 loss to drive retinoblastoma formation. In addition, epigenetic alterations have also been implicated in cooperating with RB1 mutation.
The adenomatous polyposis coli (APC) gene, which is mutated in children with familial adenomatous polyposis who are predisposed to hepatoblastoma and colon carcinoma, also controls the G1 cell cycle checkpoint. APC serves as a ubiquitin ligase that regulates the protein stability of β-catenin, which controls transcription of cyclins, including cyclin D, which in turn associates with cyclin-dependent kinase (CDK)4/6 to negatively regulate RB1 activity. Disease-associated dominant mutations of APC tend to occur in distinct regions of the gene, leading to the generation of truncated protein products, consistent with the requirement of residual or neomorphic activity of the mutant proteins. Because APC regulates the stability of many proteins—and because, in addition to the cell cycle progression, β-catenin also regulates cell adhesion and differentiation—the mechanism of tumorigenesis induced by APC mutations is thought to be pleiotropic.
In addition to mutations of genes whose products regulate cell division, cancer-causing mutations also directly dysregulate mitogenic signaling and cell growth. The most common cancer predisposition syndrome, neurofibromatosis, is predominantly caused by autosomal-dominant inheritance of inactivating mutations of the neurofibromin 1 (NF1) gene, with the less frequent form caused by mutations of neurofibromin 2 (NF2) or merlin . Children with mutations of NF1 are predisposed to the development of benign and malignant tumors, including gliomas, peripheral nerve sheath tumors, pheochromocytomas, and leukemias. NF1 is a large protein that is not completely understood but is known to regulate the activity of rat sarcoma (Ras). In turn, NF1 mutant tumors exhibit increased Ras guanosine triphosphate hydrolase activity, which stimulates the mitogen-activated protein and AKT kinase cascades that, among other effects, result in the transcription of genes involved in cell growth and stimulation of protein synthesis. Although most patients with germline NF1 mutations experience the development of neurofibromas, which harbor biallelic inactivation of the gene, only a minority of these tumors evolve to become malignant. This finding indicates that disease-associated NF1 mutations have limited transforming ability, and that additional lesions are required for carcinogenesis.
The complex relationship between cancer-causing gene mutations and their effects on tumorigenesis is also evident from mutations of ras genes themselves. Genes related to ras , including Kirsten rat sarcoma (KRAS) , neuroblastoma rat sarcoma ( NRAS) , and Harvey rat sarcoma (HRAS) , are some of the first human protooncogenes identified, based on the involvement of the corresponding viral oncogenes in the pathogenesis of rat sarcomas. However, whereas NF1 mutations stimulate Ras activity and cause neurofibromatosis, patients with inherited mutations of the ras genes instead experience the development of cardiofaciocutaneous, Noonan, LEOPARD, and Costello syndromes. Inherited mutations of the ras genes are missense activating mutations, analogous to the oncogenic viral ras mutations, yet the spectrum of tumor phenotypes in these patients is distinct. In addition to gliomas these phenotypes also include leukemias, neuroblastomas, and rhabdomyosarcomas. These differences between NF1- and ras -mutant cancer predisposition syndromes are not simply due to differences in tissue expression of the two genes, because both appear to be expressed in neuronal, mesenchymal, and hematopoietic tissues. Rather, they may reflect the developmental consequences of Ras activation as a result of the inactivation of an endogenous negative regulator as opposed to direct mutational activation of an oncogenic signaling pathway, a property that may inform the development of strategies to target these lesions therapeutically.
DNA Repair and Genome Instability
The prevention of de novo cancer-causing mutations ultimately depends on efficient DNA repair and maintenance of genome stability. Indeed, several cancer predisposition syndromes involve defective DNA repair. For example, inherited mutations of the tumor protein 53 ( TP53) tumor suppressor gene cause most cases of Li-Fraumeni syndrome, which is associated with the predisposition to sarcomas, leukemias, and breast carcinomas. The genotype-phenotype relationship of TP53 cancer-causing mutations is complex, with some mutations having apparent loss-of-function, dominant-negative, or gain-of-function properties in experimental models. p53 regulates the expression of genes involved in cell division, apoptosis, and DNA repair, and its mutation in cells leads to chromosomal instability. This process is mediated partly by direct effects of p53 on the transcription and translation of target genes, as well as via feedback interaction with its ubiquitin ligase murine double minute 2 (MDM2), which also has oncogenic effects independent of its negative regulation of p53. As a result, the dysregulation of cellular DNA damage and stress response by TP53 mutations is profoundly tumorigenic, contributing to the somatic pathogenesis of most human cancers. The p53-MDM2 feedback regulation also complicates therapeutic targeting, because inhibition of MDM2 aimed at restoring p53 tumor suppression may in effect enhance the deleterious activity of gain-of-function p53 mutant proteins.
Defective DNA damage response is also a hallmark of Fanconi anemia (FA), a cancer predisposition syndrome characterized by bone marrow failure and development of myelodysplasia, leukemia, and carcinomas. Although mutations of more than 15 different genes can cause FA, most of the known FA-mutant gene products physically interact with the components of a conserved DNA damage response mechanism, breast cancer 1 (BRCA1), ataxia telangiectasia mutated (ATM), Nijmegen breakage syndrome 1 (NBS1), and radiation-sensitive 51 (RAD51), and are required for the repair of chromosome defects that occur during homologous recombination. FA pathway–deficient cells are hypersensitive to DNA-damaging agents, and over time they accumulate deleterious gene mutations (usually segmental deletions).
Several other chromosome-breakage syndromes with cancer predisposition involve defects in closely related biochemical pathways of DNA repair of homologous recombination errors, such as ataxia telangiectasia (due to mutations of ATM ), Nijmegen breakage syndrome (due to mutations of NBS1 ), and Bloom syndrome (due to mutations of BLM ). Genes involved in nucleotide excision or transcription-coupled DNA repair can also be mutated and cause the cancer predisposition syndromes xeroderma pigmentosum and Cockayne syndrome, usually due to accumulation of ultraviolet radiation–induced mutations (typically nucleotide substitutions).
Aberrant Gene Expression
Another hallmark of cancer cells is expression of genes in inappropriate developmental states, often driven by mutations of gene products that regulate gene expression. For example, patients with inherited mutations of the Wilms tumor 1 (WT1) gene experience development of the Denys-Drash syndrome (due to loss-of-function missense mutations) or the Wilms tumor, aniridia, genitourinary anomalies, and mental retardation (WAGR) syndrome (due to gene deletions), both with predisposition to the development of bilateral Wilms tumors. In spite of years of study, the function of the WT1 protein is incompletely understood. WT1 is a key regulator of mesenchymal cell development, including kidney mesenchyme, in part through its functions as a transcriptional repressor. However, WT1 also interacts with messenger RNA (mRNA) and splicing factors, consistent with possible posttranscriptional functions in regulation of gene expression. In addition, although recessive mutations of WT1 appear consistent with its tumor suppressive functions in Wilms tumorigenesis, in other developmental and tissue contexts, particularly myeloid leukemias, WT1 can enhance cell survival and proliferation and as a result can act as an oncogene or haploinsufficient tumor suppressor gene. Such tissue-dependent phenotypes are characteristic of cancer-associated genes that affect gene expression and cell fate determination, insofar as the functional consequences of mutations depend on the spectrum of expressed genes themselves and differentiation state in general.
Tumorigenesis due to aberrant regulation of gene expression can also occur posttranscriptionally, as in the case of inherited mutations of the DICER1 gene, which predispose to the development of pleuropulmonary blastoma, cystic nephroma, and rhabdomyosarcoma. DICER1 is a ribonuclease that is required for the generation of endogenous microRNAs (miRNAs), which negatively regulate gene expression by inducing degradation and translational blockade of specific mRNAs. Cancer-associated mutations affect conserved, enzymatically required residues in DICER1 and cause defective miRNA processing, leading to increased expression of target genes. The reason inherited DICER1 mutations predispose specifically to the development of pleuropulmonary blastoma, cystic nephroma, and rhabdomyosarcoma is not understood, but it is reasonable to hypothesize that this predisposition is due to the specific requirements for miRNA-mediated gene expression in these tissues or expression of specific target protooncogenes.
Somatically Acquired Gene Mutations and Cancer Genomics
Many cancer-causing gene mutations that are inherited as part of familial cancer predisposition syndromes can also be acquired somatically in tumor cells. These mutations include most of the genes described in the preceding sections, but with notable differences in pathogenic mechanisms.
First, in many instances of somatic mutations of tumor suppressor genes, only one of the alleles is mutated, leaving the other wild-type allele intact and expressed. Such haploinsufficient mutations, as opposed to the classic “two-hit” tumor suppressor gene mutations, are thought to exert their effects through reduced tumor suppressive activities as part of tumor initiation. In addition, the functional contributions of the wild-type allele may be required for tumor maintenance in some cases. This distinction may be crucial for the development of therapeutic strategies to target haploinsufficient tumor suppressors, because restoration of tumor suppressor gene functions may be not be useful in tumors in which residual wild-type activity is required for tumor maintenance.
Second, the tumor spectrum and phenotype, and therefore the exact mechanism of tumorigenesis, are often different for the same mutation when it is developed somatically as opposed to inherited in the germline. For example, inherited mutations of WT1 cause Denys-Drash syndrome and predisposition to Wilms tumors but never leukemias, whereas somatic mutations of WT1 occur in 10% of cases of acute myeloid and T-cell acute lymphoblastic leukemias (ALLs). This difference may be due to the differences in gene expression and differentiation state of cells that are transformed by these tumorigenic mutations. The same principle is likely responsible for the nonequivalence of many other somatically acquired tumor mutations that have counterparts that occur as part of inherited developmental syndromes, such as the loss-of-function mutations of BCL6 corepressor (BCOR) that occur somatically in medulloblastomas and myeloid leukemias but cause oculofaciocardiodental syndrome without cancer predisposition when inherited in the germline.
Finally, recent whole-genome surveys have revealed that recurrence rates of somatically acquired gene mutations may be rare when considered individually but can involve coherent biologic pathways that are mutated frequently as a functional group. This functional co-occurrence is often suggested by the mutual exclusivity of the individual mutations. We review the mechanisms of tumorigenesis by these mutations, as organized below by shared biochemical activities or biologic pathways.
Genomic Rearrangements and Chromothripsis
Structural genomic rearrangements and copy number changes are particularly prevalent in adult cancers, both at the level of whole chromosomes and chromosomal segments, particularly in advanced or histologically anaplastic diseases, but they are generally somewhat less prominent in pediatric cancers. The mechanisms of their generation and tumorigenic sequelae at least in part appear to be related to the dysfunction of DNA repair and maintenance of genome stability. In particular, whole chromosome aneuploidy, often as a result of chromosomal instability due to defects in mitosis such as centrosome amplification or failure of spindle assembly, is a prevalent feature of anaplastic tumors. In some cases, such as acute myeloid leukemias, mutations of the components of the mitotic cohesin complex, including stromal antigen 2 (STAG2) , structural maintenance of chromosomes 3 and 1A (SMC3 and SMC1A) , and radiation-sensitive 21 (RAD21) , may lead to chromatin cohesion defects and aneuploidy. However, identification of such cohesin complex mutations in karyotypically normal acute myeloid leukemia (AML) raises the possibility that their tumorigenic effects rely on nonmitotic functions of the cohesin complex components.
In model systems, aneuploid cells appear to have increased rates of mutation and susceptibility to DNA damage. Noncancerous aneuploid human cells tend to be nonviable, with the exception of cells with trisomies of chromosomes 13, 18, and 21 and certain ring chromosomes. However, somatically acquired chromosomal aneuploidy is far more prevalent in tumors than in constitutional syndromes, suggesting that additional molecular changes that enable tumor cells to tolerate aneuploidy must exist. Indeed, tumors with characteristic aneuploidies often have specific additional mutations—for example, overexpression and mutational activation of cytokine receptor-like factor 2 (CRLF2) signaling in B-cell ALL (B-ALL) with trisomy of chromosome 21. Defining the molecular basis of these interactions may reveal potential opportunities to therapeutically target mechanisms that permit tolerance of aneuploidy in cancer cells.
One of the best-studied genomic aberrations in cancer cells is recurrent chromosomal translocations. These chromosomal rearrangements generally lead either to the aberrant expression of protooncogenes as a result of juxtaposition of regulatory transcriptional elements or to the generation of chimeric fusion gene products that have gain-of-function tumorigenic effects. Chromosomal translocations are frequently observed in hematopoietic cancer cells, where they lead to increased survival, growth, and malignant transformation, such as MYC-overexpressing t(8;14) translocation in Burkitt lymphomas and t(9;22) translocation generating the BCR-ABL1 fusion proteins in B-ALL and chronic myeloid leukemia. In lymphoid cells, these translocations are generated at least in part by aberrant V(D)J recombination and activation-induced deaminase activities that occur at the immunoglobulin and T-cell receptor loci undergoing somatic hypermutation. In addition, recombination of endogenous repetitive elements and DNA topoisomerase II failures also appear to cause chromosomal translocations, such as those generating mixed-lineage leukemia (MLL) fusion proteins.
The chimeric fusion proteins generated by these translocations have been found to disrupt cell differentiation and specification, along with enhanced cell survival and growth. For example, the TEL-AML1 fusion protein leads to abnormal self-renewal of hematopoietic stem cells, making them susceptible to additional mutations and ultimate transformation to B-ALL. The EWS-FLI1 fusion protein in Ewing sarcoma disrupts specification of mesenchymal progenitors, blocking cell differentiation.
By virtue of being tumor-specific, fusion gene products generated by chromosomal translocations represent attractive targets for rational therapy. Indeed, introduction of all- trans- retinoic acid and arsenic trioxide to induce the degradation of the chimeric PML-RARA fusion protein caused by the t(15;17) chromosomal translocation in the majority of cases of acute promyelocytic leukemia has revolutionized the treatment of this disease by eliminating the need for chemotherapy.
In addition to the gains and losses of whole chromosomes and their segments, cancer cells can exhibit other distinct and complex types of genomic rearrangements. In particular, some tumors such as neuroblastomas and gliomas, for example, have been found to contain short episomal DNA fragments, termed double minutes, that lack centromeres and telomeres. In neuroblastomas, these double minutes contain multiple copies of the MYCN protooncogene, leading to its high-copy amplification and overexpression. The mechanisms generating these episomal DNA fragments are not well defined but may involve recombination or transposition events involving endogenous Alu, LINE , and MER elements, or microhomology-based aberrant nonhomologous end-joining reactions.
Recently a highly complex form of genomic rearrangements, termed chromothripsis , has been described in a variety of tumors, including neuroblastomas, medulloblastomas, and osteosarcomas. This phenomenon is defined by extensive intrachromosomal rearrangements, often numbering in the thousands, of a single chromosome. It has been proposed to be caused by aberrant DNA replication and microhomology-based nonhomologous end-joining repair, as well as by damage of chromosomes trapped in micronuclei as a result of mitotic errors. The mechanisms of the generation of these complex rearrangements and their functional consequences remain to be defined but promise to reveal important insights into the mechanisms of human tumorigenesis, given their marked prevalence in distinct tumor types.
DNA Methylation, Chromatin, and the Epigenetics of Childhood Cancer
Structural abnormalities in the genomes of cancer cells seem essential for tumorigenesis, but increasingly, the contribution of aberrant epigenetic regulation to the development of human cancers in general and pediatric cancers in particular is also becoming recognized. Methylation of cytosines in DNA can cause profound changes in transcription, and methylation of CpG islands in the promoters of genes can lead to transcriptional silencing. In addition, nuclear DNA is tightly bound to histones as part of chromatin, and sequence-specific posttranslational modification of histones, including acetylation, methylation, and ubiquitination, have all been shown to modulate DNA accessibility and transcription. Consequently, the transcription of individual genes depends both on the degree of DNA compaction and accessibility, as well as on the individual chemical modifications of histones surrounding specific genes.
DNA Methylation
Methylation of cytosines in DNA is a key regulator of mammalian cell development, and changes in DNA methylation have long been known to be associated with human cancer. For example, loss of normal DNA methylcytosine imprinting patterns on chromosome 11 causes Beckwith-Wiedemann syndrome, a developmental disorder that predisposes to the formation of hepatoblastomas and Wilms tumors. Indeed, abnormal patterns of cytosine methylation are frequently observed in tumor cells, and recently mutations of enzymes that control DNA methylation have been found in AML and myelodysplastic syndromes.
The de novo DNA methyltransferase 3A (DNMT3A) is recurrently mutated in a large fraction of cases of AML. The mutations tend to be missense and heterozygous, involving an evolutionarily conserved DNA-binding interface of DNMT3A, consistent with a possible gain-of-function effect that presumably alters locus-specific DNA methylation. Loss-of-function deletions and nonsense mutations of methylcytosine dioxygenase 2 (TET2) are also observed in AML and myelodysplastic syndromes. TET2 functions by hydroxylating methylcytosine, and these mutations are thought to lead to the loss of DNA hydroxymethylcytosine, although whether this is carcinogenic in itself or transforms cells indirectly via its effects on histone modifications and DNA demethylation remains to be determined. Consistent with this notion, the CpG island methylator phenotype, which is a key factor in the tumorigenesis of adult colorectal carcinomas, is yet to be definitively established in tumors with defects in DNA methylation.
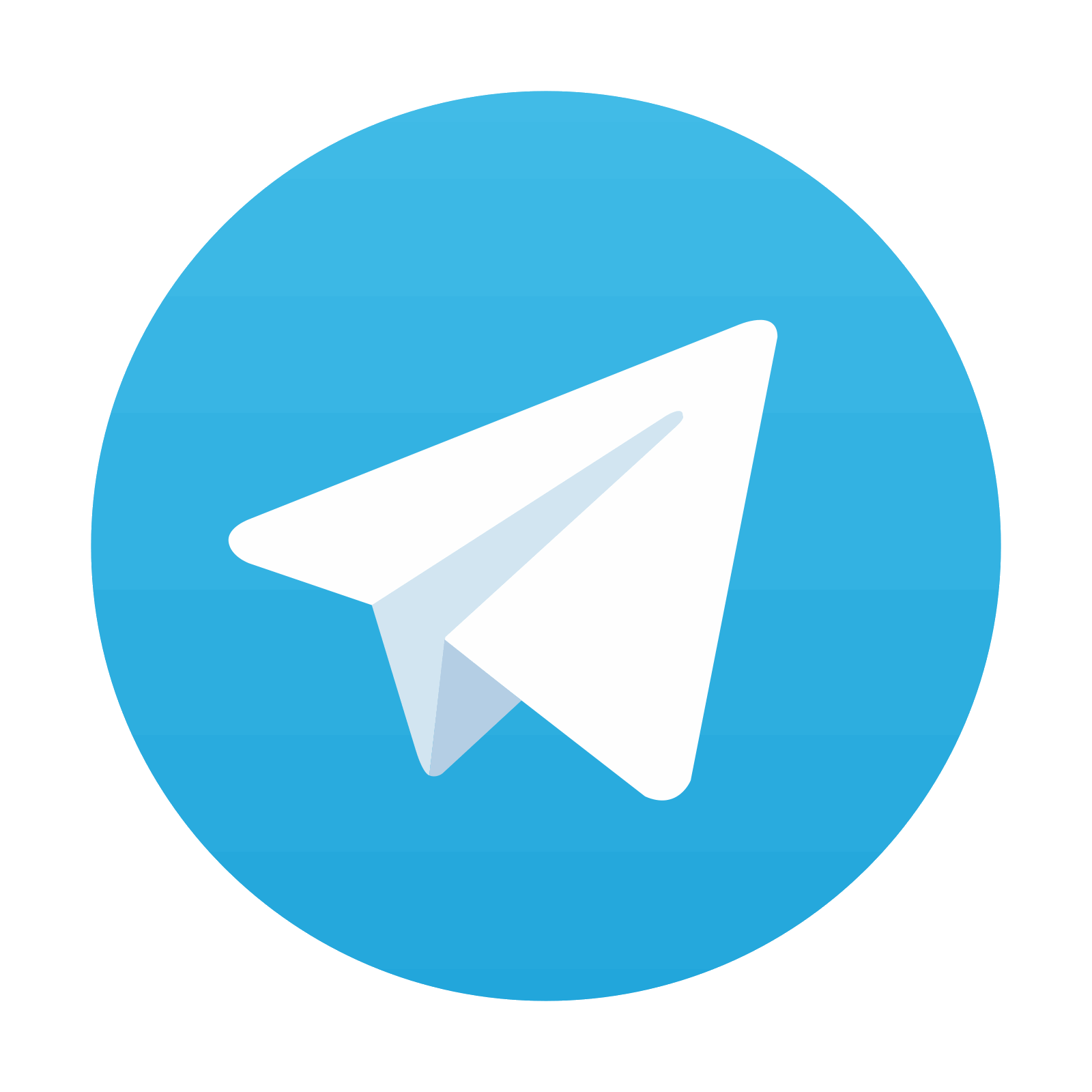
Stay updated, free articles. Join our Telegram channel
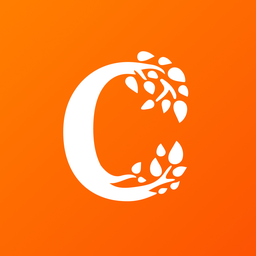
Full access? Get Clinical Tree
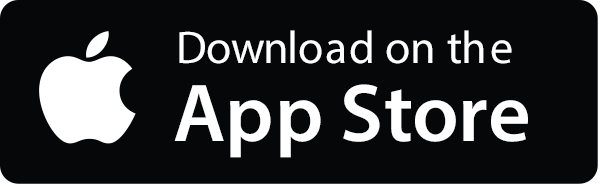
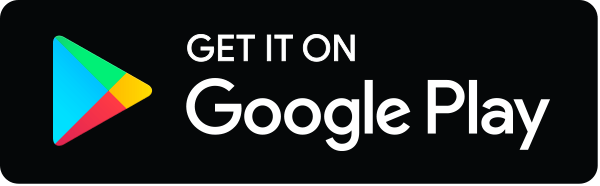