After nearly two decades of struggle, gene therapy for hemoglobinopathies using vectors carrying β or γ-globin gene has finally reached the clinical doorsteps. This was made possible by advances made in our understanding of critical regulatory elements required for high level of globin gene expression and improved gene transfer vectors and methodologies. Development of gene editing technologies and reprogramming somatic cells for regenerative medicine holds the promise of genetic correction of hemoglobinopathies in the future. This article will review the state of the field and the upcoming technologies that will allow genetic therapeutic correction of hemoglobinopathies.
Key points
- •
Hemoglobinopathies are the most common genetic defects worldwide. Hematopoietic stem cell (HSC) transplant, although curative, is limited by the availability of matched donors.
- •
Genetic modification of autologous HSC overcomes the availability of donors (every patient is their own donor) and immunological side effects (graft versus host disease/graft rejection).
- •
Critical determinants unique to gene therapy for hemoglobinopathies are erythroid-lineage and developmental stage-specific high levels of transgene expression and pretransplant conditioning that will allow 10%–20% gene-modified HSC chimerism.
- •
Scientific insights into regulation of the globin gene locus and improvements in gene transfer technology have led to development of β-/γ-globin based additive gene therapy a clinical reality.
- •
Clinical trials with β-/γ-globin lentivirus vectors are now open at multiple sites and transfusion independence following gene therapy has been reported in 1 patient with β-thalassemia.
- •
Promising new technologies such as induced pluripotent stem cells and genome editing can usher in a new era in the field of gene therapy.
Introduction
Over the last 2 decades significant advances have been made in gene therapy for hemoglobinopathies. Gene therapy has exploited the ability of retrovirus (RV) vectors, which are equipped with the machinery to reverse transcribe their RNA into complementary DNA (cDNA) and integrate this cDNA into the host cell genome to deliver therapeutic genes into cells. Inherited hematopoietic disorders are potentially targetable, because hematopoietic stem cells (HSC) can be readily isolated from bone marrow or mobilized peripheral blood, manipulated ex vivo, and transplanted back using current tools and knowledge of bone marrow transplant technology. The initial vectors used for gene therapy were derived from the murine Moloney leukemia virus (MLV), a simple retrovirus belonging to the γ-retrovirus family, and were termed RV vectors. Despite initial successes in several immunodeficiency disorders, limitations of the RV include their inability to infect the quiescent nondividing HSC, hematopoietic malignancies from inadvertent integration of these vectors near cellular proto-oncogenes, and their activation by the viral promoter enhancer in the vector long terminal repeat (LTR) regions. For hemoglobinopathies, an additional limitation is their inability to carry the large cargo of the globin gene and its regulatory elements, required for high-level expression. These limitations prompted the development of human immunodeficiency virus (HIV)–based vectors. HIV belongs to the lentivirus (LV) family of retroviruses and hence these vectors are termed LV vectors. LV have several advantages for gene therapy for hemoglobinopathies. They differ from RV in their ability to enter an intact nucleus and integrate into nondividing cells, and hence they transduce HSC efficiently. Their self-inactivating (SIN) design, which removes all viral transcriptional machinery, and their ability to carry a large cargo makes them ideally suited for gene therapy for hemoglobinopathies. Since the first use of gene therapy for adenosine deaminase (ADA) deficiency in 1990, generational changes have made the vector design more effective and safe, advanced molecular tools have made it easier to study the insertional effects of gene transfer. Gene therapy has been shown to be effective in correction of many immunodeficiency disorders such as X-linked severe combined immunodeficiency (X-SCID), Wiskott-Aldrich syndrome (WAS), and chronic granulomatous disease (CGD). However, gene therapy for hemoglobinopathies has a unique set of challenges.
Hemoglobinopathies have the additional challenge of requiring high levels of expression of β-/γ-globin genes for therapeutic correction. Identification of critical regulatory elements needed for high expression of β-globin transgene has made gene therapy for β-hemoglobinopathies (sickle cell disease [SCD] and β-thalassemia) a feasible option. More recently, encouraging results from the first successful gene therapy for a patient with hemoglobin E-β-thalassemia in a French trial has opened up gene therapy as a potential definitive treatment option for patients with β-hemoglobinopathies. Trials with different iterations of the β-globin and γ-globin genes in SIN LV to treat thalassemia or SCD are beginning in Italy and 4 centers in the United States (New York, Memphis, TN; Cincinnati, OH; and Los Angeles, CA).
Because expression of increased levels of fetal hemoglobin (HbF) significantly ameliorates the phenotype of both SCD and β-thalassemia, critical elements involved in γ-globin repression in postnatal life have been identified, which has opened up new gene therapy approaches to increase endogenous γ-globin production without the need to add the globin genes and the large locus control region regulatory elements.
Even more promising is the development of gene editing approaches. The design of zinc finger nuclease (ZFN)–mediated repair was the first such approach. This approach was soon superseded by transcription activator–like effector nucleases (TALENs) and, more recently, by the CRISPR/Cas technology, both facilitating efficient gene editing to correct the genetic defect in situ in cells with high specificity and efficiency. Once this technology is refined, it will allow genetic correction of the β-hemoglobinopathies in situ in HSC, without the need for gene insertion therapy. This article reviews the advances in gene therapy for hemoglobinopathies, with specific reference to β-thalassemia and SCD.
Introduction
Over the last 2 decades significant advances have been made in gene therapy for hemoglobinopathies. Gene therapy has exploited the ability of retrovirus (RV) vectors, which are equipped with the machinery to reverse transcribe their RNA into complementary DNA (cDNA) and integrate this cDNA into the host cell genome to deliver therapeutic genes into cells. Inherited hematopoietic disorders are potentially targetable, because hematopoietic stem cells (HSC) can be readily isolated from bone marrow or mobilized peripheral blood, manipulated ex vivo, and transplanted back using current tools and knowledge of bone marrow transplant technology. The initial vectors used for gene therapy were derived from the murine Moloney leukemia virus (MLV), a simple retrovirus belonging to the γ-retrovirus family, and were termed RV vectors. Despite initial successes in several immunodeficiency disorders, limitations of the RV include their inability to infect the quiescent nondividing HSC, hematopoietic malignancies from inadvertent integration of these vectors near cellular proto-oncogenes, and their activation by the viral promoter enhancer in the vector long terminal repeat (LTR) regions. For hemoglobinopathies, an additional limitation is their inability to carry the large cargo of the globin gene and its regulatory elements, required for high-level expression. These limitations prompted the development of human immunodeficiency virus (HIV)–based vectors. HIV belongs to the lentivirus (LV) family of retroviruses and hence these vectors are termed LV vectors. LV have several advantages for gene therapy for hemoglobinopathies. They differ from RV in their ability to enter an intact nucleus and integrate into nondividing cells, and hence they transduce HSC efficiently. Their self-inactivating (SIN) design, which removes all viral transcriptional machinery, and their ability to carry a large cargo makes them ideally suited for gene therapy for hemoglobinopathies. Since the first use of gene therapy for adenosine deaminase (ADA) deficiency in 1990, generational changes have made the vector design more effective and safe, advanced molecular tools have made it easier to study the insertional effects of gene transfer. Gene therapy has been shown to be effective in correction of many immunodeficiency disorders such as X-linked severe combined immunodeficiency (X-SCID), Wiskott-Aldrich syndrome (WAS), and chronic granulomatous disease (CGD). However, gene therapy for hemoglobinopathies has a unique set of challenges.
Hemoglobinopathies have the additional challenge of requiring high levels of expression of β-/γ-globin genes for therapeutic correction. Identification of critical regulatory elements needed for high expression of β-globin transgene has made gene therapy for β-hemoglobinopathies (sickle cell disease [SCD] and β-thalassemia) a feasible option. More recently, encouraging results from the first successful gene therapy for a patient with hemoglobin E-β-thalassemia in a French trial has opened up gene therapy as a potential definitive treatment option for patients with β-hemoglobinopathies. Trials with different iterations of the β-globin and γ-globin genes in SIN LV to treat thalassemia or SCD are beginning in Italy and 4 centers in the United States (New York, Memphis, TN; Cincinnati, OH; and Los Angeles, CA).
Because expression of increased levels of fetal hemoglobin (HbF) significantly ameliorates the phenotype of both SCD and β-thalassemia, critical elements involved in γ-globin repression in postnatal life have been identified, which has opened up new gene therapy approaches to increase endogenous γ-globin production without the need to add the globin genes and the large locus control region regulatory elements.
Even more promising is the development of gene editing approaches. The design of zinc finger nuclease (ZFN)–mediated repair was the first such approach. This approach was soon superseded by transcription activator–like effector nucleases (TALENs) and, more recently, by the CRISPR/Cas technology, both facilitating efficient gene editing to correct the genetic defect in situ in cells with high specificity and efficiency. Once this technology is refined, it will allow genetic correction of the β-hemoglobinopathies in situ in HSC, without the need for gene insertion therapy. This article reviews the advances in gene therapy for hemoglobinopathies, with specific reference to β-thalassemia and SCD.
Rationale
Disorders of β-globin such as β-thalassemia and SCD are among the most common monogenic defects in the world. Based on World Health Organization worldwide estimates, about 275,000 babies with SCD and 56,000 babies with β-thalassemia are born each year. Improved supportive care has prolonged the survival of these patients. However, there is still significant long-term mortality and morbidity associated with these diseases.
In β-thalassemia, mutations affecting every step in β-globin expression have been reported, from initiation of transcription to posttranslational modification. These defects either result in decreased β-globin production (β + ) or an absence of β-globin production (β 0 ). The decreased β-globin synthesis in β-thalassemia results in excess of alpha globin, leading to intracellular precipitation of excess unbound alpha globin chains in erythroid precursors. This condition results in red cell membrane damage, early cell death, and ineffective erythropoiesis. At present, lifelong regular transfusions and iron chelation is the mainstay in the management of thalassemia. Despite aggressive iron chelation, iron overload leading to tissue damage and organ toxicity is the leading cause of morbidity and mortality in these patients. Inadequate transfusions lead to massive erythroid hyperplasia, extramedullary hematopoiesis, splenomegaly, and failure to thrive.
SCD is caused by a point mutation (A-T) in the sixth codon of the β-globin gene. This genetic defect results in defective β-globin, which polymerizes in the deoxygenated state leading to change in shape of an otherwise round, doughnut-shaped, flexible red blood cell (RBC) into a hook/sickle-shaped, inflexible RBC that obstructs microvessels and has reduced survival from repeated cycles of sickling and unsickling. SCD is characterized clinically by varying degree of anemia, and episodic vaso-occulsive crisis leading to mutiorgan damage and premature death. Medical management options currently available for SCD include supportive management of vaso-occlusive crisis, long-term transfusions to avoid or prevent recurrence of severe complications of SCD such as stroke or acute chest syndrome, and HbF induction with hydroxyurea. Despite improvement in the supportive care, the estimated life expectancy for patients with SCD is significantly foreshortened; the median age at death was 42 years for men and 48 years for women with SCD.
At present, allogeneic hematopoietic stem cell transplant (HCT) is the only established definitive curative option for patients with β-thalassemia and SCD. In both these diseases, the overall survival following matched sibling donor (MSD) HCT is excellent in children, with disease-free survival rates greater than 80%, and a graft failure rate of about 10%.
However, most patients do not have a human leucocyte antigen (HLA)-matched sibling. In SCD, it is estimated that less than 14% have a matched sibling and, in the United States, only 30% of African Americans have an identifiable matched unrelated donor (MUD) for HCT ( Fig. 1 ). Over the last decade, MUD HCT have increasingly been used for management of thalassemia and SCD. Results from the Italian multicenter trial showed an excellent overall survival and thalassemia-free survival of 97% and 80%, respectively, in low-risk groups. However, in the high-risk groups, the results were less encouraging; overall survival and thalassemia-free survival were considerably lower at 65% and 54%, respectively. Most of this mortality was attributed to grade II to IV graft-versus-host disease (GVHD) or graft failure.
In addition, there is poor acceptance of allogeneic HCT as a treatment options among patients with SCD and fewer than 400 transplants have been performed for this disease, because the HCT-related side effects are not acceptable to this patient population (see Fig. 1 ). Considering all these limitations with allogeneic HCT, gene transfer using autologous stem cells can potentially cure both thalassemia major and SCD, and could overcome the problems in allogeneic HCT, especially lack of available donors and immunologic side effects such as GVHD or graft rejection.
Prerequisites for successful gene therapy in β-hemoglobinopathies
The following requirements need to be met for successful gene therapy for β-globinopathies:
- 1.
High-efficiency gene transfer and high HSC engraftment
- 2.
Position independent or consistent expression independent of the site of integration
- 3.
High level expression of the β-/γ-globin gene expression
- 4.
Erythroid lineage–specific and developmental stage-specific expression of the transferred β-globin gene
- 5.
Safe expression with little or no risk of insertional mutagenesis/oncogenesis
Understanding the developmental switch of β-globin and its regulation in postnatal life
In humans, β-like gene cluster is on chromosome 11 and it consists of 5 functional genes: ε, Gγ, Aγ, δ, and β. During different stages of human development, timed switch in expression occurs within this gene cluster. In fetal life, the γ-globin gene (resulting in HbF; α2γ2) is the predominant gene expressed in β-globin locus and β-globin gene expression is repressed. However, after birth, expression of γ-globin gene decreases to negligible levels, with concomitant increase in β-globin expression, which decreases HbF with a corresponding increase in HbA (α2β2). This process of switching from γ- to β-globin is complex, and it is regulated by multiple transcription factors such as GATA1, BCL11A, KLF1, MYB, and SOX6, and chromatin modification by histone deacetylase 1 and 2 (HDAC 1 and 2). The current interest to increase γ-globin expression stems from some important observations. In both SCD and β-thalassemia, hereditary persistence of HbF (HPFH) leads to milder disease phenotype. As a result, pharmacologic measures to induce HbF have resulted in clinical improvement. An in-depth understanding of the role of these regulatory elements in γ-globin gene silencing during postnatal life can help design targeted interventions to reactivate endogenous γ-globin expression.
The regulation of β-globin is also complex, and elements apart from the β-globin promoter are needed for high levels of expression of the β-globin gene. A regulatory element of 40 to 60 Kb upstream of the β-globin gene, known as the locus control region (LCR), is a strong erythroid-specific enhancer that is necessary for high levels of expression of the β-globin gene. The LCR is extremely sensitive to DNase 1 in erythroid cells, with 5 DNase hypersensitive (HS) sites, HS1 to HS5 ( Fig. 2 ). Of the 5 HS regions, HS2 was critical for the position independent activity of the LCR, and HS3 was an important determent of its enhancer activity. Similar to the LCR for the β-globin locus, HS40, a DNase-hypersensitive site was identified as an alpha globin enhancer 40 Kb upstream of the alpha globin locus acts as an alpha globin enhancer.
Introduction to gene therapy
Many viral and nonviral vectors have been tried for gene transfer into cells. Nonviral vectors include plasmids or naked DNA that require physical means such as electroporation to enter cells. The gene transfer efficiency is best with virus vectors, because they have evolved over millions of years to be able to readily enter cells. In addition, physical techniques are too harsh for fragile cells such as HSC. Although nanoparticles that can carry the genetic material in a phospholipid scaffold are a promising proposition, a lot of work is still needed to improve their efficiency and specificity.
Most of the viral vectors in preclinical studies and in clinical trials are derived from Retroviridae, a family of viruses that can permanently integrate into cellular genomes. Other viral vectors include adenovirus, herpes simplex virus, vaccinia, pox virus, and adeno-associated virus, but lack the integration apparatus. The viruses in the Retroviridae family that are currently used in clinical trials or in advanced preclinical testing include retrovirus (RV), LV, and foamy virus (FV) vectors. These viral vectors are bioengineered viral particles in which the genetic elements needed for pathogenicity and replication are removed and are replaced by the cellular transgene of interest. By using these viral vectors, clinicians could use the viral machinery to enter cells and accomplish the delivery and permanent integration of the transgene of interest into human genome, thus correcting the underlying genetic defect ( Fig. 3 ). A synopsis of common terminology used in gene therapy and comparison of different characteristics of the commonly used gene therapy vectors in hemoglobinopathies are given in Tables 1 and 2 respectively.
Viral vector | Bioengineered genetically disabled viral particles devoid of the components responsible for pathogenic potential but that act as carriers of the therapeutic transgene of interest |
LTR | Long terminal repeats are identical sequences at the ends of RVs: LTR contains U3, R, and U5 regions. The U3 region has strong promoter and enhancer activity and is used by the virus to reverse transcribe and integrate the genetic material into the host genomes |
SIN | Self-inactivating vector design is used to improve safety. Deletion of the U3 region in the 3′ LTR deletes the TATA box and enhancer and abolishes the LTR promoter and enhancer activity. This deletion does not affect vector integration |
Transgene | Human cellular gene (transgene) carried by the vector |
Promoter | Cellular promoter needed for transgene expression |
Enhancer | Cellular enhancers are short segments of DNA that enhance the transcription of the cellular gene, unlike promoters they may not be in close proximity to the gene |
Insulator | Boundary elements flanking the gene cluster of interest; they protect the transgene from silencing by surrounding heterochromatin. Insulators also have enhancer blocking activity |
Process | |
Titers | Vectors are made from different plasmid systems in packaging cells. The vector yield or “titer” is measured as the number of particles per milliliter |
Transduction efficiency | Percentage of cells that have been genetically modified by the vector. The higher the transduction (gene transfer) efficiency the better the outcome |
Insertion site | The site in the host chromosome where the vector integrates the transgene with minimal viral elements. The preference of insertion sites vary among different integrating vectors |
Vector copy | Number of transgene/vector copies integrated per cell of interest |
Gamma RV Vectors | LV Vectors | FV Vectors | |
---|---|---|---|
Genus | Retroviridae | Retroviridae | Retroviridae |
Viral genome | RNA | RNA | RNA → cDNA |
Pathogenicity | Derived from pathogenic murine leukemia virus | Derived from pathogenic HIV virus | Derived from nonpathogenic, nonhuman FV |
Cell division requirement | Yes | No | Yes |
Packaging limitation | Up to 5 Kb, unstable with large transgene cassettes | Up to 7 Kb | Up to 9 Kb, large transgene cassettes |
Transduction efficiency | High in dividing cells | High in dividing and nondividing cells | Efficient entry in dividing and nondividing cells. However, integration occurs in dividing cells |
Integration site | Preferentially near transcription start sites (60%–70%) | Preferentially within transcribed genes (60%–70%) | Integration into nongenic regions is preferred approximately 60% of the time. However, integrants that occur in gene-rich regions have the same profile as RV |
Safety in clinical trials | Leukemia in X-SCID and WAS trials | No overt malignancy seen after 2–6 years | No human clinical data |
MDS/monosomy 7 in CGD trial | Clonal expansion of HMGA2 in one thalassemia patient, that has now decreased | ||
Experimental genotoxicity data | High risk for insertion oncogenesis | Safer than RV. Safety high with self-inactivating design and use of cellular promoters and enhancers | Safer integration profile than RV and LV |
Main advantages | Longest human data and experience | Better safety profile than RV vectors | Nonpathogenic, can carry a large cargo and may be safer than RV and LV |
SIN RV vector design may address safety concerns | Can stably carry larger cargo Efficiently transduce nondividing HSC | ||
Main disadvantage | Clinically significant risk of insertion oncogenesis Lower capacity Does not transduce non-dividing cells | Might induce dysregulation/disruption of cellular genes, albeit at low frequency | Still not in human trials |
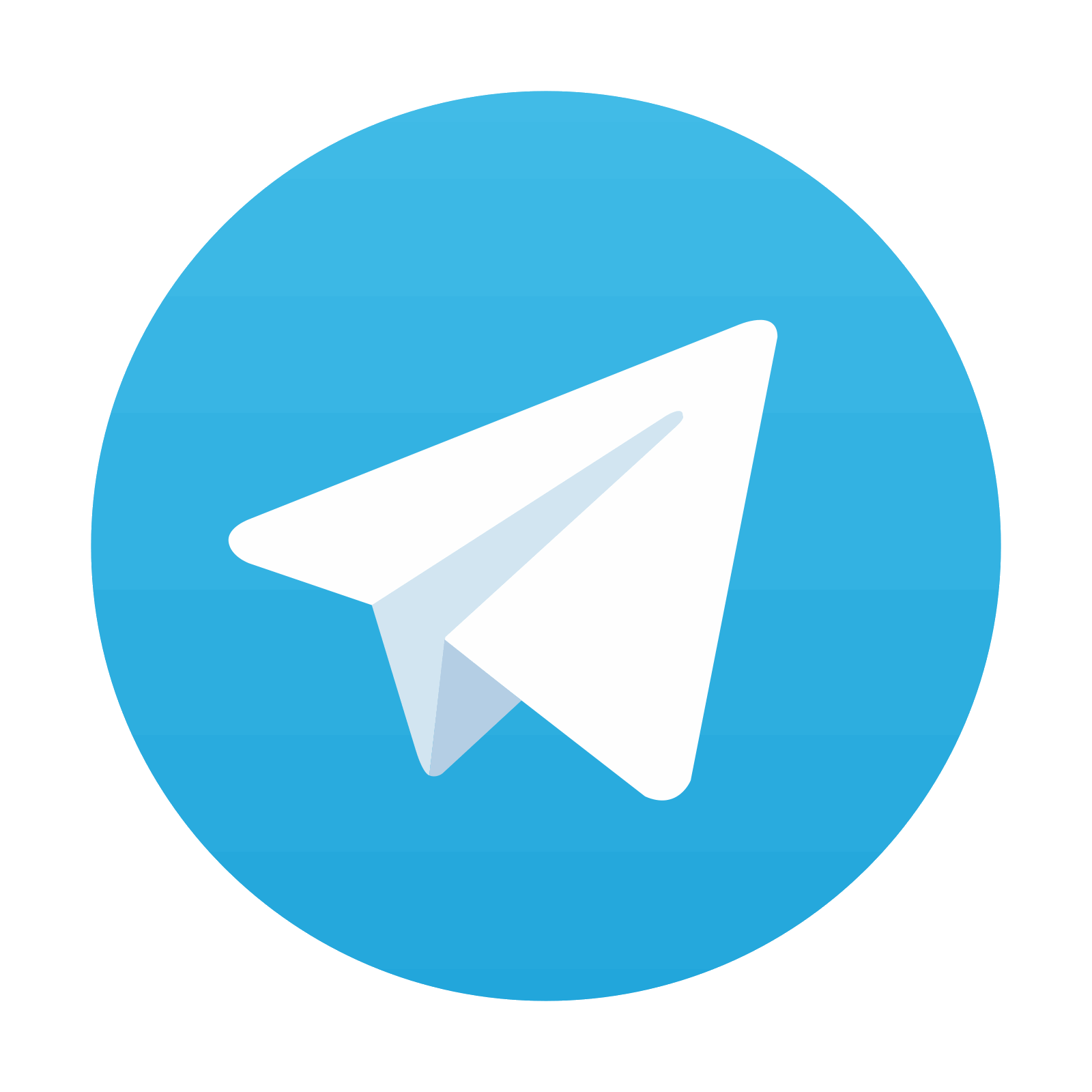
Stay updated, free articles. Join our Telegram channel
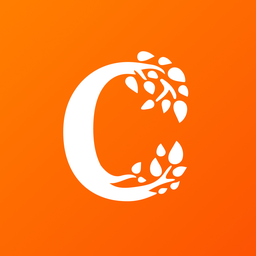
Full access? Get Clinical Tree
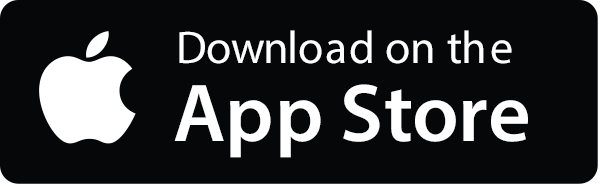
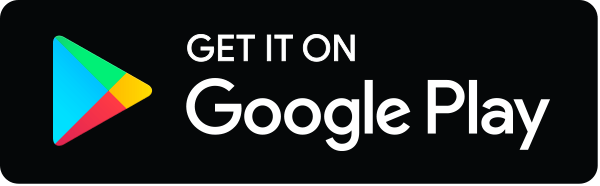
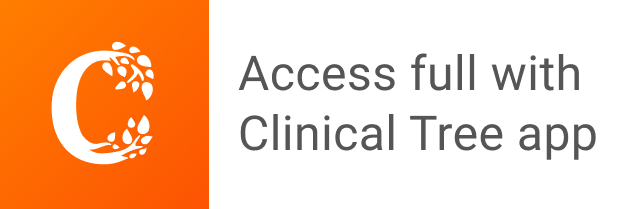