8 Functional Imaging
The surgical management of brain tumors requires detailed functional mapping of cortical regions around a tumor. Traditional intraoperative methods are electrical cortical stimulation (ECS) or electrocorticography (ECoG). Although these methods are considered gold standards, they are not helpful for presurgical planning. Preoperative localization of functionally intact brain tissue helps guide neurosurgical planning and limits the region of resection, allowing for improved long-term patient morbidity and neurologic function. Various techniques are available to preoperatively and noninvasively map functional brain organization, such as positron emission tomography (PET), functional magnetic resonance imaging (fMRI), and magnetoencephalographic imaging (MEGI). This chapter reviews each of these methods for noninvasive functional brain imaging.
Surgery is often used in the management of patients with brain tumors or pharmacologically resistant epileptic foci. However, there is a trade-off between the margin of excision used to ensure complete removal and the potential loss of function that may arise as a consequence of removing normal surrounding brain tissue. There are several invasive approaches used in neurosurgery to define eloquent areas of the cortex prior to surgical excision. One approach is to perform electrophysiological mapping of the cortex in the awake patient at the time of the operation. A second approach routinely used prior to surgery is the Wada test, in which the predominant side of the brain used for language and memory is identified by the invasive sequential injection of sodium amytal (which transiently stops the brain from working) into each of the two main blood vessels supplying different sides of the brain, during standard neuropsychological testing.
Preoperative mapping techniques can result in a precise estimation of the location of functional areas in relation to a tumor or epileptic focus, therefore reducing surgical risk. Although traditional brain imaging methods such as magnetic resonance imaging (MRI) and computed tomography (CT) provide detailed knowledge of the structure of the brain, these methods do not tell us about brain function. Several studies have shown that considerable variability exists in anatomic identification of functional brain areas, even by experts.1 This variability can be significantly reduced by functional brain mapping methods.
Pearl
• Preoperative functional imaging can result in a precise estimation of the location of functional areas in relation to a tumor, therefore reducing surgical risk.
Pearl
• Positron emission tomography (PET), functional magnetic resonance imaging (fMRI), and magnetoencephalographic imaging (MEGI) are the most popular preoperative functional brain imaging methods.
Functional brain imaging allows us to look inside the brain of humans in “real time” and appreciate the neural mechanisms underlying behavior, rather than just observing the consequences of these effects in lesion studies. Functional brain imaging has revealed the neural basis for several behaviors, such as how we are able to make sense of and integrate large amounts of dynamic information in our environment, understand language, learn new skills, and remember important facts. Preoperative functional brain imaging techniques can be integrated with neuro-navigational systems to provide intraoperative guidance to the surgical team.2–8 Preoperative functional brain imaging can provide significant information for determining the surgical approach, screening of patients for awake craniotomy, planning for intraoperative mapping, and the limits of resection.
The earliest studies of preoperative mapping in brain tumors were PET studies of sensorimotor, language, and visual areas. Since its advent in 1990, fMRI has quickly become widely available and has also been extensively used before surgery. More recently, MEGI has become more widely available for planning and guiding tumor surgery and can also measure tumor infiltration.
Positron Emission Tomography
Positron emission tomography is a nuclear medicine technique requiring a tracer compound of physiological interest that is introduced into the body. This tracer is labeled with radioactive isotopes of atoms (such as oxygen, carbon, or nitrogen) that emit positrons. Once a positron is emitted from the nucleus of the atom, it will speed in an unpredictable direction, and before it traverses a distance of less than a millimeter, it will collide with one of the electrons in its environment. As a result of this collision, the positron and electron will be annihilated and converted to high-frequency photons in the gamma frequency range that will fly with equal speed in diametrically opposite directions. The energy of these photons is sufficient to propel them without distortion clear through the brain tissues and the skull to the surface of the head. Scintillation detectors arranged outside the head consist of a crystal coupled to a photomultiplier tubes or avalanche photodiodes.
When a photon hits a crystal, visible light is emitted. The light interacts with the cathode plate inside the photomultiplier tube, causing emission of electrons, which in turn interact with a series of dynodes that successively multiply their number such that each photon results in a sufficiently amplified electrical pulse to be handled by large-scale timers and counters. The precise timing and counting of pulses generated by a series of such detectors is the basis for determining where each positron emission originates. Specifically, it is the simultaneous or coincident detection of the pair of photons moving in approximately opposite direction that is the basis of PET reconstruction. Photons that do not arrive in temporal pairs, that is, within a timing-window of a few nanoseconds, are ignored.
Reconstruction algorithms enable tomographic reconstruction images of the distribution of positron-emitting radioactivity in the body. Using statistics collected from tens of thousands of coincidence events, a set of simultaneous equations for the total activity of each parcel of tissue can be solved by a number of techniques, and, thus, a map of radioactivity as a function of location for parcels or bits of tissue (also called voxels) may be constructed and plotted. The PET scans are often read alongside CT or MRI scans, giving co-registered anatomic and metabolic information. Modern PET scanners are now available with integrated CT, or more recently MRI scanners. Because these multimodality scans can be performed in immediate sequence during the same session, with the patient not changing position between the two types of scans, the two sets of images are more precisely registered; that is, they are in alignment, so that areas of abnormality on the PET imaging can be more perfectly correlated with anatomy on the CT or MR images.
Positron emission tomography can be used to trace the biological pathway of any compound in living humans provided it is radiolabeled with a PET isotope. Radioisotopes or radionuclides used in PET scans are typically isotopes with short half-lives such as carbon-11 (~20 minutes), nitrogen-13 (~10 minutes), oxygen-15 (~2 minutes), fluorine-18 (~110 minutes), or rubidium-82 (~1.27 minutes). These radionuclides are incorporated either into compounds normally used by the body, such as glucose (or glucose analogues), water, or ammonia, or into molecules that bind to receptors or other sites of drug action. Such labeled compounds are known as radiotracers. Using radiotracers, the specific processes that can be probed with PET are virtually limitless, and radiotracers for new target molecules and processes are continuing to be synthesized. There are already dozens of compounds in clinical use and hundreds used in research. By far the most commonly used radiotracer in clinical PET scanning is fluorodeoxyglucose (FDG; also called fludeoxyglucose), an analogue of glucose that is labeled with fluorine-18.
Functional brain imaging with PET is based on an assumption that areas of high radioactivity are associated with brain activity. What is actually measured indirectly is the flow of blood to different parts of the brain, which is, in general, believed to be correlated, and has been measured using the tracer oxygen-15. However, because of its 2-minute half-life, O-15 must be piped directly from a medical cyclotron for such uses, which restricted PET scans to a few centers. More recently, however, the development of ligands such as [11C] raclopride and [18F] fallypride for dopamine D2/D3 receptors, [11C]McN 5652 and [11C] DASB for serotonin transporters, or enzyme substrates (e.g., 6-fluoro-Dopa [F-Dopa] for the AADC enzyme) permit the visualization of neuroreceptor pools in the context of a plurality of neuropsychiatric and neurologic illnesses.
Thus, highly accurate measurements of blood flow, volume, glucose, oxygen and protein metabolism, neuroreceptor and transmission system function, and blood–brain barrier permeability can be achieved with PET. However, regional cerebral blood flow (rCBF) is the favored functional brain imaging technique because it can be measured quickly using oxygen-15–labeled water with a half-life of 123 seconds, which allows for repeat measurements in the same patient.
A typical PET functional image is obtained by subtraction of a scan obtained during a baseline condition from a scan obtained during an active condition. Studies with PET have brought to light the fact that metabolic changes accompanying brain activation do not appear to follow the time-honored notion of a close coupling between blood flow and oxidative metabolism of glucose. Changes in blood flow appear to be accompanied by changes in glucose utilization that exceed the increase in oxygen consumption, suggesting that oxidative metabolism of glucose may not supply all of the energy demands encountered transiently during brain activation. Rather, glycolysis alone may provide the energy needed for transient changes. The above issues confound inferences about neuronal activity from PET. Nevertheless, successful functional localization accomplished by activation studies in which PET scanning has been used has been shown to correlate well with the results of intraoperative cortical stimulation mapping.9–12
Functional Magnetic Resonance Imaging
Functional magnetic resonance imaging (fMRI) in general may refer to any MRI technique to measure physiological function. However, the term typically refers to techniques developed in the early 1990s that exploit a phenomenon called blood oxygen level dependence (BOLD) response. The BOLD response is based on the fact that properties of water molecules in the brain change slightly between areas that are near blood, with its oxygen exhausted relative to those near freshly oxygenated blood. Local increase in energy requirements arising as a consequence of neuronal firing is largely met through an increase in oxygen-based metabolism, with the increased demand for oxygen being delivered seconds later by an increase in the local blood flow. Changes in the oxygenation level of the blood, therefore, occur as a consequence of neuronal activity, and the magnitude of change in signal intensity can be used as an indirect measure of excitatory input to neurons. The MRI signal can be made sensitive to the amount of oxygen in hemoglobin, and changes in blood oxygen content at sites of brain activation can be detected. Thus, increased neuronal activity causes a BOLD signal and is reflected as a small increase in signal intensity on the MRI scan. Activation patterns in working human brain can be mapped with high temporal and spatial resolution using fMRI based on the BOLD effect.13
• It is important to note that when using both fMRI and PET, it is not the neuronal response that is monitored directly but the “surrogate” combination of a metabolic and hemodynamic response.
Although BOLD activations have been demonstrated with many different MRI schemes, most fMRI work is done with single-shot echo planar imaging (EPI), in which a single slice of the brain can be acquired in 30 to 100 ms. The key advantage of single-shot EPI is that the speed of data collection renders the images insensitive to physiological motions that would create artifacts in standard images (e.g., pulsatile flow). Similar to PET, fMRI activation maps are obtained by statistical methods that are equivalent to subtraction of images obtained during baseline conditions from images obtained during active conditions. A standard mapping protocol in fMRI is a block design experiment, where blocks of the stimulus or task are presented typically for 20 to 30 seconds, alternating with periods of rest, or a control condition. The control task is chosen carefully such that it activates all of the neural processes common to the stimulus task, with the exception of the cognitive process of interest. By subtracting the brain regions recruited during the performance of the control task from the brain regions recruited during the test condition, the areas of the brain having activity associated specifically with the cognitive process of interest can be identified.
An alternative experimental approach is to present stimuli as isolated brief events separated in time so that the individual response to single events can be identified. The principal advantage of this event-related approach is that it avoids the potential confounding factors of habituation or fatigue, which may arise in a block design as a consequence of the presentation of repeated identical stimuli. Although this approach can preserve much of the temporal information in the hemodynamic response, it is not often used in functional brain mapping studies. Typically, changes in signal intensity are on the order of a few percent; hence the need for effective statistical methods to extract meaningful signals.
The hemodynamic response (occurring over seconds) is much slower than the neuronal response (occurring over tens to hundreds of milliseconds). Although there is typically a delay of 4 to 6 seconds between the onset of the task and the peak latency of the hemodynamic response, the temporal resolution of fMRI as a technique would not be compromised if the shape of the hemodynamic response function was fixed and was simply convolved with the time course of the taskinduced neural activity. However, there is some evidence that characteristics of the hemodynamic response may vary between different individuals, between different brain regions, with the nature of the task, or in the presence of disease. The unknown potential variability in the latency of the hemodynamic response limits precise interpretations of the magnitude and the temporal resolution of the signal. The latter problem, however, does not generally limit uses of fMRI as a “mapping” technique. For such experiments, the time course of the response is itself not the most critical issue.14
Several studies have reported the effectiveness of fMRI in correctly identifying the localization of the main motor strip or language area preoperatively in patients with lesions near these eloquent regions and for preoperative evaluation of patients with intracranial tumors, and have correlated sensorimotor and language activation with ECS.15–20 Patients with tumors close to eloquent sensorimotor or language areas have been assessed with motor, sensory, and language paradigms. Motor and sensory paradigms are more effective than language paradigms. Many activation maps often yield high or adequate quality and are useful in the decision to operate, as well as in the decision of surgical approach and extent of resection.21–24
Functional MRI is more prone to artifacts caused by visualization of the draining veins, which may explain the more cranial and lateral activation, whereas PET depicts capillary perfusion changes and therefore shows activation closer to the parenchyma. Although a variety of studies have discussed its potential, there is still some doubt about the localization accuracy, which might be impaired, at least in part, by the low signal-to-noise ratio (SNR), susceptibility artifacts, and draining veins. Fluorodeoxyglucose-PET and fMRI have good correspondence. Advantages of fMRI over PET are higher spatial temporal resolution, shorter examination time, wider availability, and lack of exposure to radioactivity. When evaluating fMRI, however, caution is needed because of multiple sources of artifacts. Correction of distortions and motionrelated artifacts is important. Positron emission tomography has a higher SNR and lesser susceptibility to artifacts, and is amenable to patients with MRI contraindications. Pathophysiological factors might cause neurovascular uncoupling and facilitate artifactual findings with fMRI in patients with direct tumor infiltration, neovascularity, cerebrovascular inflammation, and arteriovenous malformation (AVM)-induced hemodynamic effects. Lesion-induced neurovascular uncoupling causing reduced fMRI signal in perilesional eloquent cortex, in conjunction with normal or increased activity in homologous brain regions, may simulate hemispheric dominance and lesion-induced homotopic cortical reorganization.
Pearl
• Advantages of fMRI over PET are higher spatial and temporal resolution, shorter examination times, wider availability, and no exposure to radioactivity.
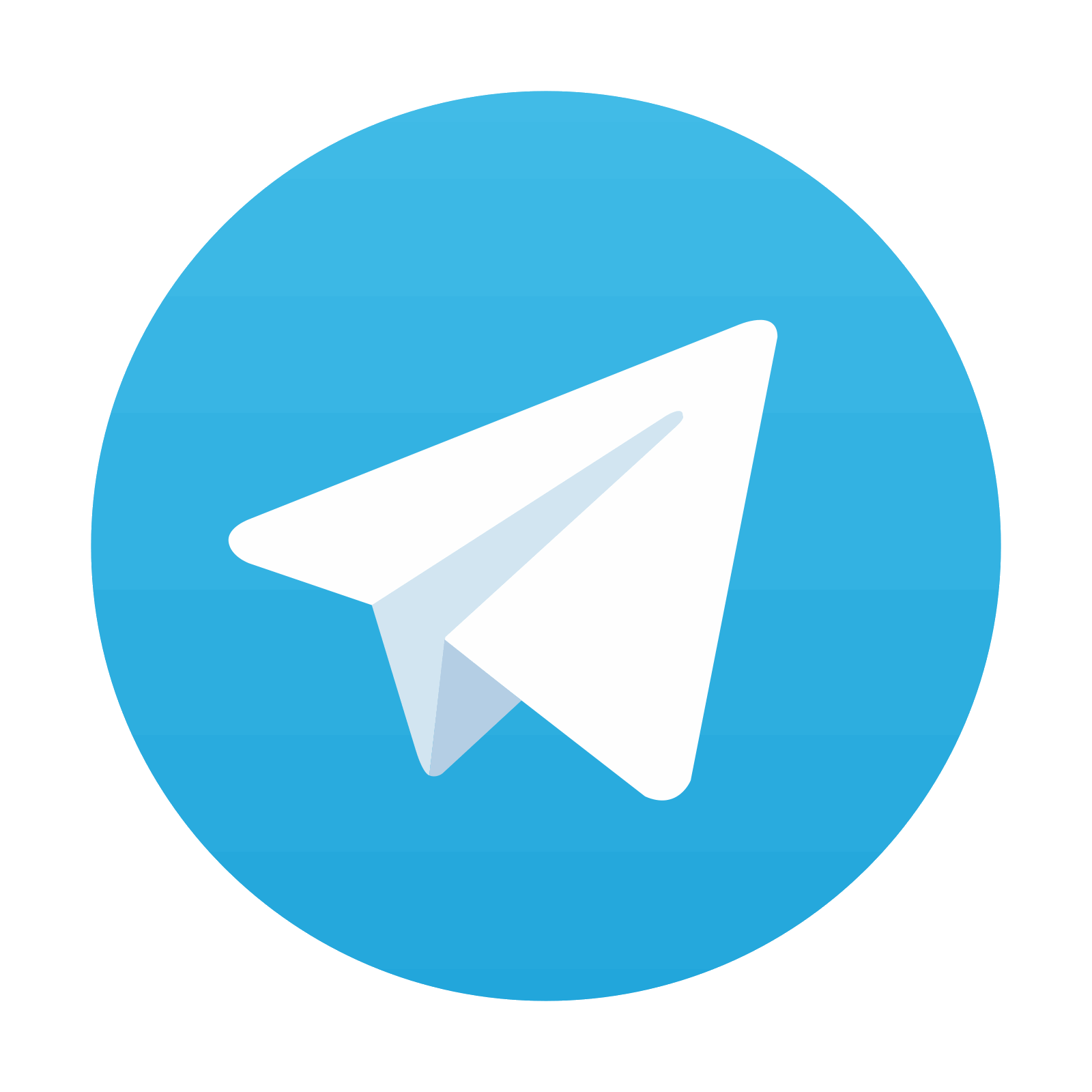
Stay updated, free articles. Join our Telegram channel
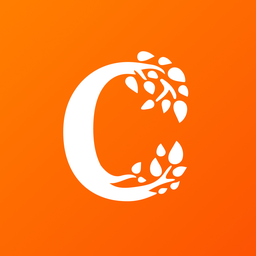
Full access? Get Clinical Tree
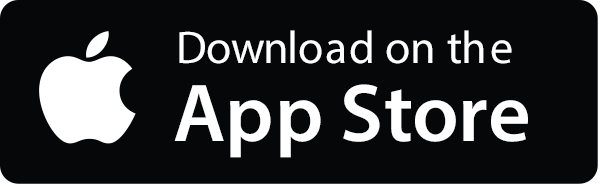
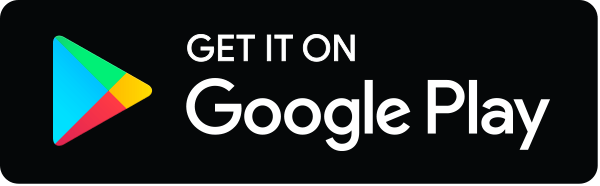