16 Fractionated Radiotherapy
Radiotherapy is commonly used in the management of malignant and selected benign brain tumors. The majority of patients requiring radiotherapy are treated with fractionated external-beam photon beams, delivering 1.8 to 2.0 Gy per day, generated by linear accelerators (LINACs). Modern innovations in radiation treatment delivery technology (Fig. 16.1) have revolutionized radiation oncology treatments for brain tumor patients, and include near-rigid patient immobilization devices (Fig. 16.2), computed tomography (CT) and magnetic resonance imaging (MRI)-based simulation to allow for multimodal imaging-based target delineation, incorporation of on-board image guidance systems to ensure daily verification of the patient position prior to delivery, and precise beam shaping using multileaf collimators (MLCs) that also allow for modulation of the beam intensity during delivery (known as intensity modulated radiotherapy [IMRT]).1 The potential benefits of these innovations include increasing tumor control by allowing safe dose-escalation, and decreasing risk of late radiation toxicity by minimizing the volume of brain exposed to collateral radiation dose.
Radiobiology and Physics
Radiation produces highly reactive free radicals in tissues. These free radicals damage nuclear DNA, resulting in reproductive cell death during cellular division or apoptotic cell death in response to the DNA injury.2 There are three categories of radiation damage: (1) lethal damage, which is irreversible and leads to cellular death; (2) sublethal damage, which can be repaired unless additional sublethal damage is added; and (3) potentially lethal damage, which can be modified by environmental conditions.2 The apparent radioresponsiveness of tissue depends on the inherent sensitivity of cells, the kinetics of the cell population, the microenvironment such as oxygen levels, and radiation parameters such as type of radiation, absorbed dose, and fractionation. Absorbed dose is the amount of energy deposited per unit mass at a point in tissue (measured in units of joules per kilogram, commonly referred to as a gray [Gy]).
Although photons (X-rays or gamma rays) are the most commonly used form of therapeutic radiation, charged particle therapies such as protons or other heavy particles like carbon or helium ions have also been used for the treatment of specific central nervous system (CNS) tumors such as chordomas.3 Dose for charged particle beam therapy is typically quoted in cobalt gray equivalents (dose normalized for the relative biological effectiveness [RBE] of the particle versus cobalt gamma irradiation). For protons, the RBE is close to that of photons at 1.1, and can be considered biologically equivalent. The major benefit of a proton beam over a photon beam stems from the Bragg peak energy deposition that allows for sharp dose gradients at interfaces with critical structures, as the dose abruptly falls off at a depth in tissue specific to the energy of the proton beam.4,5 This characteristic has resulted in protons being recognized as a standard of care, for the delivery of ultrahigh-dose radiation treatments for selected tumors of the brain and spine.3,6,7 For example, historically, patients with clival chordoma and chondrosarcoma have been treated with protons with the aim of delivering doses in excess of 70 Gy, the reason being that the proximity of these tumors to critical structures like the brainstem, optic nerves, and chiasm limits the dose (tolerance typically 50 to 60 Gy depending on the fractionation) that can be safely delivered with conventional nonconformal radiation techniques.8
However, recent advances in photon beam delivery with IMRT and image-guided radiotherapy (IGRT) have improved the ability to deliver these high doses safely, and offer cost-effective and accessible alternatives to proton beam therapy.3 Intensity modulated radiotherapy with subcentimeter leaf width MLCs can create steep dose gradients between the tumor and organs at risk (OAR) to permit the high-dose deposition (Fig. 16.3), and IGRT provides the security that the dose is delivered with submillimeter accuracy.9 The drawback of IMRT lies in the increased exposure to the surrounding normal brain tissues to low-dose radiation (integral dose) as compared to protons.5 This characteristic suggests protons may be justified for children with brain tumors, as it is assumed that less dose exposure to the healthy brain tissue would translate into a lower risk of a second malignancy and potentially other late effects such as neurocognitive impairment.
Controversy
• Particle beam accelerators are more expensive and complex than X-ray–based LINACs, and the cost-benefit analysis of charged particle therapy versus image-guided IMRT remains a major topic for debate.
Fig. 16.2a–c Three head frames commonly used for radiation delivery. (a) A patient with an invasive head frame receiving LINAC-based stereotactic radiosurgery (SRS). (b) A patient being fitted with a near-rigid relocatable head frame (aimed to replace the use of invasive frames), which is based on a head fixation device coupled to a bite block attached to a vacuum device via tubing that provides suction for the bite block to affix to the hard palate (Aktina system). (c) A nonrigid thermoplastic mask that is typically applied for fractionated daily radiotherapy and hypofractionated stereotactic radiotherapy when coupled to an image guidance system.
Unlike protons, charged particles such as carbon ions are truly distinct from photons or protons, as the radiobiological effect is considerably larger than photons due to a greater linear energy transfer (LET) and higher efficiency in generating lethal DNA injury.10 Although the physical properties of particle beams make them attractive for CNS irradiation, particle beam accelerators for heavy ion therapy are considerably more expensive and complex than X-ray linear accelerators, and there are still no data to support superiority with respect to clinical outcomes. Although protons are increasingly becoming available, carbon ions are available in only a few centers worldwide.
Radiation Fractionation
Radiation effects are manifest as cell attrition due to apoptosis or reproductive cell death. The brain is unique in that the normal parenchymal cell populations (neuronal, glial, and vascular) are either static or slowly dividing. Consequently, the clinical manifestations of radiation side effects within the normal brain usually do not appear until months to years after radiation is completed (i.e., late or delayed reaction). Normal CNS parenchyma is very sensitive to the size of individual doses or fractions of radiation, reflecting a large capacity for radiation repair with fractionated treatment. Tumor cells, for the most part, have less capacity for sublethal and potentially lethal damage repair, and are spared to a lesser degree at conventional fraction sizes compared with normal tissue. Other advantages to fractionation include the possibility of tumor cells undergoing re-assortment into more radiosensitive phases of the cell cycle and reoxygenation of hypoxic cells between fractions, as hypoxic cells are more radioresistant. Thus differences in radiosensitivity between normal and tumor cells can be exploited by fractionation to improve the therapeutic ratio.2 Most contemporary radiation treatments are fractionated on a once-a-day basis, Monday to Friday, over a period of 5 to 6 weeks (25 to 30 treatments), with total doses of 50 to 60 Gy.
Hyperfractionation and accelerated fractionation involve delivering multiple (usually two to three) fractions per day to allow an increase in the total dose, a reduction in late side effects (hyperfractionation), or a shorter overall treatment time to reduce the effects of tumor repopulation during treatment (accelerated fractionation).2 Accelerated and hyperfractionation schemes have been explored in clinical trial settings for malignant supratentorial and brainstem gliomas and CNS lymphoma, but no significant clinical advantage over daily fractionation to conventional doses has been generally noted.11 Conventional hypofractionation utilizes a larger dose per fraction, such as 3 to 5 Gy, to enable the delivery of therapeutic doses over shorter periods of time, and has been demonstrated to provide similar survival benefits when compared with conventionally fractionated treatments for patients with poor prognosis glioblastoma.12 The use of altered fractionation schemes in other types of primary brain tumors has not been extensively explored.
Evidence is emerging that there is a separate mechanism of radiation response linked to the tumor microvasculature as a critical step in tumor death.13,14 Work done on tumor response to single large doses of radiotherapy (8 to 10 Gy) suggests that damage to the tumor microvasculature takes place within 6 hours via activation of the acid sphingomyelinase (ASM) pathway.13 Tumor cell death then follows secondary to vascular collapse. At the cellular level, membrane alterations caused by only high doses of ionizing radiation (> 8 Gy) result in ASM enzyme hydrolyzing sphingomyelin into ceramide, which then acts as an apoptosis messenger.13 Because ASM is present 20-fold more in endothelial cells than in epithelial and tumor cells, this is a common pathway of apoptosis in the endothelium. This work supports the hypothesis that damage to tumor endothelial cells is strongly linked to tumor cell damage and is critical in the radiation response although this has yet to be definitely proven. This research may explain why radiosurgery has been so effective in tumor control despite sacrificing the potential benefits of re-assortment and reoxygenation that are present with longer fractionated course. The advent of modern radiation delivery systems has allowed the use of hypofractionated stereotactic radiotherapy (HSRT),15 and HSRT with fraction sizes of ≥ 6 Gy is emerging as a common therapeutic modality for metastatic brain tumors.16
Stereotactic radiosurgery (SRS) refers to a single high dose of radiation delivered to a target localized in three-dimensions with technology that allows for submillimeter precision. The aim with SRS is to target only the visible tumor based on imaging, and to limit normal brain tissue exposure. Normal brain tissue is much less tolerant of high-dose radiation, with the consequence of radiation-induced edema and radiation necrosis.17,18 Consequently, SRS is traditionally delivered with the use of an invasive head frame and a dedicated Gamma Knife (Elekta, AB, Stockholm, Sweden) or LINAC unit employing either multiple converging static beams or arcs.1 The combination of rigid head immobilization, high precision collimation, and multiple beam trajectories afforded by these dedicated units ensures precision delivery and rapid dose falloff, maximizing brain sparing outside the target to be treated. The ability to spare the brain even with these dedicated units declines as lesions exceed 4 cm in size, and thus SRS is typically restricted to the treatment of smaller and well-circumscribed (noninvasive) lesions.
The role of SRS in the primary management of glioma is limited to small recurrent tumors.19 The routine use of SRS boost in the primary treatment of malignant glioma has been investigated in a randomized trial which concluded no survival benefit.20 Stereotactic radiosurgery is more often applied to brain metastases under 4 cm, and for selected small benign brain tumors such as acoustic neuroma and meningioma. In order to circumvent the size restriction inherent to SRS, HSRT is emerging as an alternative to SRS in the management of multiple tumor types and indications. Essentially, the approach refers to delivering two to five fractions of high dose per fraction radiation daily (> 5 Gy/fraction), with patients immobilized in a noninvasive head frame/mask. The benefits lie in that the size restriction associated with single-fraction SRS is relaxed by allowing for repair in between the fractions, and the lower dose per day is inherently less damaging both acutely and in the long term. Although it is necessary to reduce normal brain tissue exposure, the normal brain tissue can take a higher dose when exposed in this limited fractionated approach. Thus, rates of radiation necrosis are potentially reduced,16 and larger or infiltrative lesions may be treated without the same risk of toxicity as SRS.16
As an example, the experience of using 30 Gy in five fractions as a salvage regimen for 24 patients with recurrent glioblastoma multiforme (GBM) in combination with bevacizumab has been reported.19 Beyond the encouraging median overall survival rate of 12.5 months and a 1-year overall survival rate of 54%, no patient experienced radiation necrosis. It is likely that the bevacizumab yielded protective effects, mitigating the development of radiation necrosis; however, the fractionated approach of the radiation may also have helped lessen the risk of radiation necrosis.
In another series specific to brain metastases, 30 patients with surgically resected brain metastases were treated with 27.5 to 35 Gy in five fractions (median 30 Gy) to the postoperative surgical cavity using HSRT. The median planning target volumes (PTVs) were large, ranging from 24 to 35 cc and irregular, which would be prohibitive with single-fraction SRS due to the risk of radiation necrosis. The rates of local control were 79% and 100% at 1 year in those with no prior whole-brain radiation therapy (WBRT; 20 patients/21 cavities) and prior WBRT groups (10 patients/11 cavities), respectively. Importantly, no radiation necrosis was observed in the 21 surgical cavities treated in the no prior WBRT group. In a review of HSRT versus SRS for intact metastases, similar outcomes were noted for HSRT,21 and another series indicated superior outcomes despite larger volumes treated when comparing HSRT to SRS.16 As evidence supporting clinical equivalence continues to emerge, the advantages of HSRT16 include the use of noninvasive head frames, less restriction by size of the target volume and proximity to OAR, and the ability to use conventional modern LINACs instead of specialized dedicated units, which suggests LINAC-based HSRT may become a modality of choice compared to traditional SRS.
Dose Selection
For primary infiltrative brain tumors, the most typical approach is to radiate with conventional fractionation of 1.8 to 2.0 Gy/day up to 50 to 60 Gy. This is the standard dose for the radical treatment of individuals with low- or high-grade glioma, either alone or in combination with chemotherapy. In the case of elderly patients or poor performance status patients with glioblastoma, results with conventionally fractionated radiotherapy have been disappointing,22 and alternate radiation schedules have been investigated.12 Shorter courses of radiation at 34 to 40 Gy in 10 to 15 fractions (2.67–3.4 Gy/d), designed to deliver similar biologically equivalent doses to 54 to 60 Gy in 30 fractions, have been shown to yield similar survival and toxicity rates as compared to more protracted schedules.12,23
When dealing with patients with a very poor performance status and an expected short survival, a more palliative approach to radiation may be justified. Short radiation schedules ranging from 20 to 30 Gy in five to 10 fractions can be given with the goal of temporizing the disease in the short-term, and minimizing radiation-induced side effects.23
For benign tumors such as meningioma, schwannoma, and craniopharyngioma doses of 50 to 54 Gy in 1.8- to 2.0-Gy fractions per day are commonly used.24 If the tumors are small and amenable to SRS, this is a viable alternative with equivalent local control rates and more convenient for the patient. Use of HSRT is increasing in benign tumors but does not yet have the long track record of SRS.
Chemical Modifiers of Radiation Effect
Hypoxic cells have been demonstrated in vitro to exhibit up to a threefold decrease in radiosensitivity and may represent a radioresistant cell population, manifested clinically as tumors that have areas of central necrosis. Hypoxic cell sensitizers, high concentrations of oxygen (hyperbaric oxygen, carbogen), and hemoglobin modifiers have been utilized to address this issue.2 Dose-limiting toxicity, poor drug delivery to tumor, and reoxygenation during standard fractionated treatment may account for the lack of therapeutic benefits observed in clinical trials to date. Halogenated pyrimidines are thymidine analogues, which are incorporated into the DNA of actively cycling cells and increase radiosensitivity in vitro. Clinical trials of these agents in malignant glioma have not resulted in improved survival.
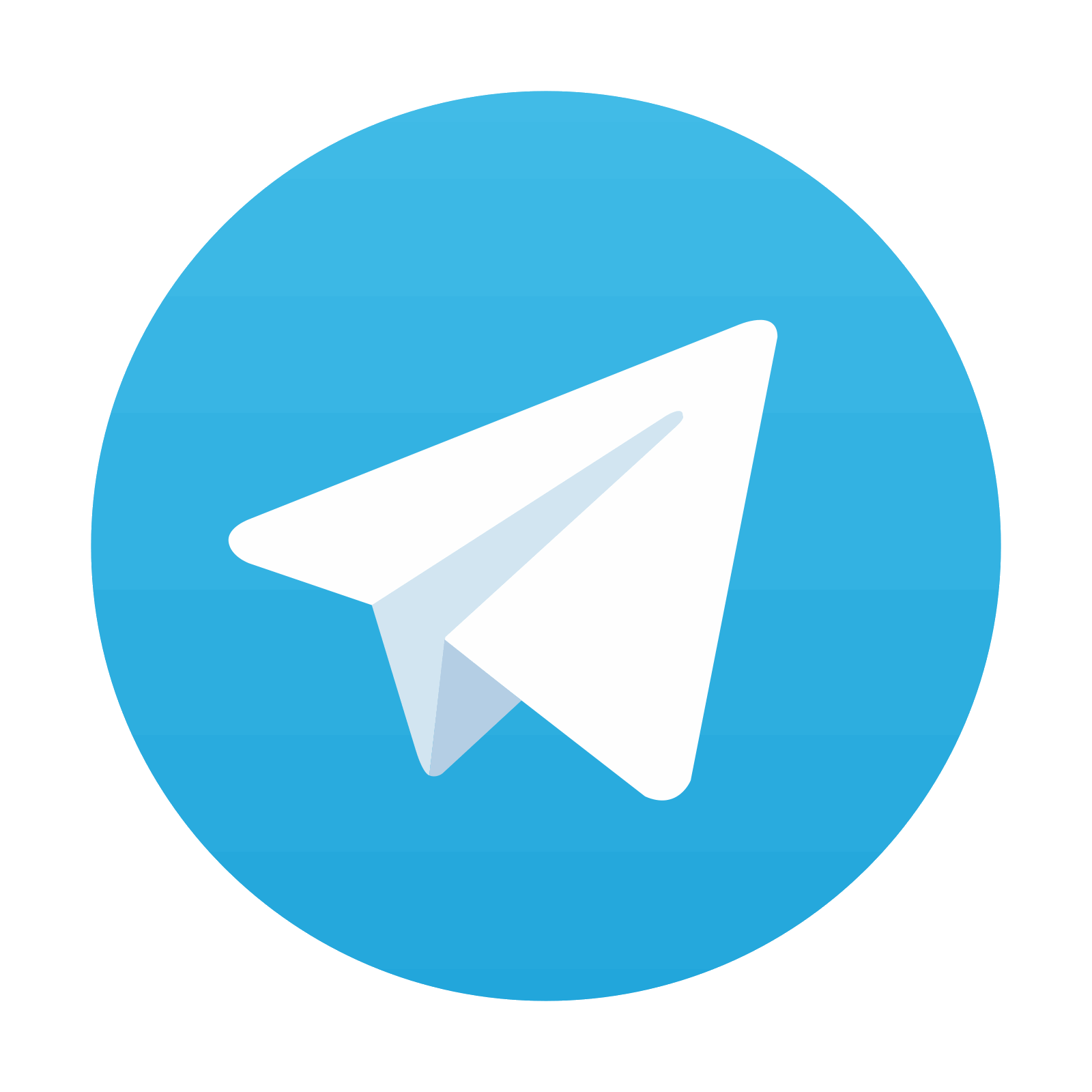
Stay updated, free articles. Join our Telegram channel
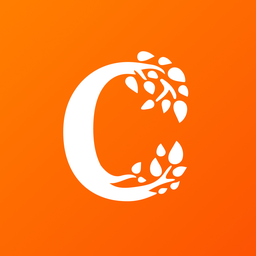
Full access? Get Clinical Tree
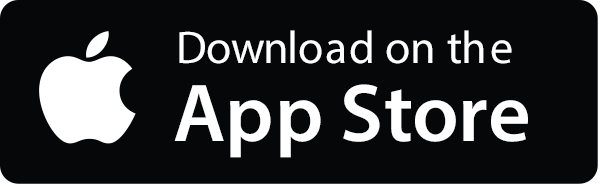
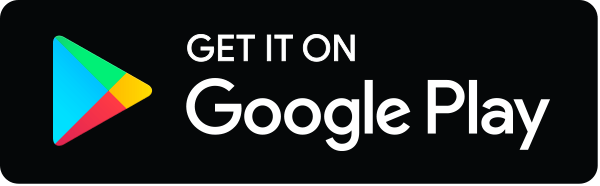