Calcium (Ca), phosphorus (as phosphate [HPO 4 2− ]), and magnesium (Mg) are essential nutrients that are indispensable for the structural integrity of the body and for the function of each of its cells. The genetic and physiological mechanisms that regulate normal mineral homeostasis and bone development, composition, and strength from the prenatal period through adolescence are complex. Lists some of the many genes that direct these processes. Figs. 9.1 and 9.2 schematically depict the factors that regulate calcium and phosphate homeostasis, respectively.
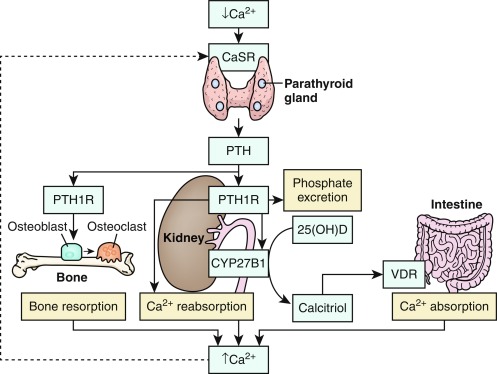
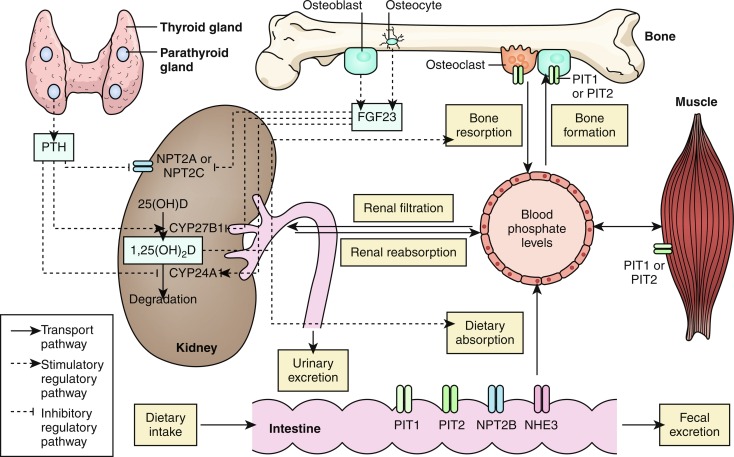
Calcium
Calcium is present in extracellular fluids, cell cytoplasm, and bone and is essential for the function of every cell in the body. Together, calcium and phosphate form the hydroxyapatite crystal [Ca 10 (PO 4 ) 10 (OH) 2 ] of bone; hydroxyapatite accounts for 65% of bone weight and provides its mechanical and weight-bearing strength and also serves as a reservoir for calcium that may be quickly needed for homeostatic and functional purposes. Although 99% of total body calcium is present in the slowly exchangeable, deeply deposited skeletal crystal, it is the rapidly exchangeable 1% of body calcium in recently accumulated surface bone and in the vascular, extracellular, and intracellular (soft tissues) spaces with which it is in equilibrium that modulates gene expression, intercellular communication and intracellular signal transduction, neural transmission, cell-to-cell adhesion, clotting, striated, smooth and cardiac muscle contraction, cardiac rhythmicity, enzyme action, synthesis and secretion of endocrine and exocrine factors, fertilization, and cellular proliferation and apoptosis. Approximately 50% of total serum calcium is bound to albumin and globulin; 5% is complexed/chelated to citrate, phosphate, lactate, bicarbonate, and sulfate; and 45% is present as biologically active and closely regulated ionized extracellular calcium (Ca 2 + ). Serum total and ionized calcium concentrations are related to levels of albumin, creatinine, parathyroid hormone (PTH), phosphate, and serum pH. For every 1 g/dL decline in the serum concentration of albumin below 4 g/dL, the total serum calcium level declines by 0.8 mg/dL. The measured Ca 2 + level is dependent upon the serum pH (normal adult range 1.1–1.3 mmol/L at pH 7.4); increase in alkalinity (higher pH) raises calcium binding to albumin, thus decreasing the plasma Ca 2 + value, whereas acidic changes (lower pH) decrease binding, thereby increasing the Ca 2 + concentration. The relationship between pH and Ca 2 + is best described by an inversely S-shaped third-degree function. The serum concentration of Ca 2 + is maintained within narrow limits by an integrated system involving the plasma membrane Ca 2 + sensing receptor (CaSR); PTH and its receptor (PTH/PTH-related protein [PTHrP]-1R); the thyroidal parafollicular C cell product calcitonin and its receptor; and the vitamin D hormone system acting upon the intestinal tract, bone, and kidney. Thus absorption of calcium in the small intestine is enhanced by 1,25-dihydroxyvitamin D3 (calcitriol); the proximal renal tubular reabsorbs calcium filtered through the renal glomerulus; PTH and calcitriol mobilize calcium from the hydroxyapatite apatite crystal, and calcitonin secreted by the parafollicular C cells of the thyroid gland suppresses PTH secretion (see Fig. 9.1 ).
The intracellular concentration of cytosolic free Ca 2 + (approximately 100 nM) is 10,000-fold less than that in serum and extracellular fluid (ECF), a gradient maintained by exchange of Ca 2 + across the cell’s plasma membrane and across the membranes of intracellular structures. Within the cell, Ca 2 + is primarily (99%) stored within the endoplasmic reticulum (sarcoplasmic reticulum in muscle cells) and mitochondria, as well as within endosomes, lysosomes, the Golgi apparatus and secretory granules and bound to the interior of the cell plasma membrane from which sites it can be released by chemical signals, for example, inositol-1,4,5-trisphosphate (IP 3 ). Inositol-1,4,5-trisphosphate acts upon IP 3 receptors (encoded by ITPR1 –see Table 9.1 ) located in the membrane of the endoplasmic reticulum to effect rapid egress of Ca 2 + from storage, thereby quickly increasing intracellular Ca 2 + levels. Ca 2 + serves as a “second messenger” signal transducer that controls many cellular activities, including gene transcription, cell division and growth, cell movement, and secretion of synthesized products. Ca 2 + enters the cell through transmembrane protein “pores,” such as voltage-gated and store-operated calcium channels. Voltage-gated Ca 2 + channels are active in cells that are electrically excitable, such as cardiac, skeletal, and smooth muscle cells, neurons, gastric mucosa, and pancreatic β cells and that “open” in response to depolarization of the plasma membrane, permitting rapid influx of Ca 2 + into the cell
1,25(OH) 2 D 3 | 1,25-Dihydroxyvitamin D 3 (calcitriol) | LRP | Low-density lipoprotein receptor-related protein |
24R,25(OH) 2 D 3 | 24,25-Dihydroxyvitamin D 3 | μFE | Microfinite element |
25OHD 3 | 25-Hydroxyvitamin D 3 (calcidiol) | MAPK | Mitogen-activated protein kinase |
AA | Amino acids | MARRS-BP | Membrane-associated rapid response steroid-binding protein |
Acan | Aggrecan | MATN3 | Matrilin 3 |
ADHR | Autosomal dominant hypophosphatemic rickets | M-CSF | Macrophage colony-stimulating factor |
AF | Activating function | MEPE | Matrix extracellular phosphoglycoprotein |
AKT | see PKB | Mg 2 + | Magnesium |
AMP | Adenosine monophosphate | MITF | Microphthalmia-associated transcription factor |
APC | Adenomatous polyposis coli | MMP | Matrix metalloproteinase |
ARHR | Autosomal recessive hypophosphatemic rickets | MRI | Magnetic resonance imaging |
ASARM | Acidic serine aspartate-rich MEPE-associated motif | Na + | Sodium |
ATP | Adenosine triphosphate | NAD | Nicotinamide adenine dinucleotide |
ATPase | Adenosine triphosphatase | NADPH | Nicotinamide adenine dinucleotide phosphate |
BAP | Bone alkaline phosphatase | NF-κB | Nuclear factor-κB |
BMAD | Bone mineral apparent density (~ volumetric BMD) | NFATC1 | Nuclear factor of activated T cells, cytoplasmic, calcineurin-dependent 1 |
BMC | Bone mineral content | NHERF | Sodium-hydrogen exchanger regulatory factor |
BMD | Bone mineral density | NPT2 | Sodium/phosphate transporter 2 |
BMP | Bone morphogenetic protein | NTX | Amino-terminal cross-link telopeptide of collagen type I |
BMU | Basic multicellular unit | OMIM | Online Mendelian Inheritance in Man |
BRU | Bone remodeling unit | OPG | Osteoprotegerin |
BTT | Bone transmission time | OSCAR | Osteoclast-associated receptor |
Ca 2 + | Calcium—ionized | OSTM1 | Osteopetrosis-associated transmembrane protein 1 |
CaMK | Ca 2 + /calmodulin-dependent protein kinase | P3H1 | Prolyl 3-hydroxylase-1 |
CART | Cocaine amphetamine-regulated transcript | PHEX | Phosphate-regulating gene with homologies to endopeptidases on the X chromosome |
CaSR | Calcium sensing receptor | PICP | Procollagen type I carboxyl-terminal propeptide |
CGRP | Calcitonin gene-related peptide | PINP | Procollagen type I amino-terminal propeptide |
Cl − | Chloride | PIIINP | Procollagen type III amino-terminal propeptide |
CNP | Natriuretic hormone type C | PI3K | Phosphoinositide-3-kinase |
COMP | Cartilage oligomeric protein | PKA | Protein kinase A |
CREB | Cyclic AMP-responsive element binding protein | PKB | Protein kinase B (AKT) |
CTX | Carboxyl-terminal cross-link telopeptide α1 chain of type I collagen (see ICTP) | PLC | Phospholipase C |
DAG | 1,2-Diacylglycerol | PO 4 | Phosphate (HPO 4 2− ) |
DBP | Vitamin D binding protein | PPARγ | Peroxisome proliferator activator receptor gamma |
DMP | Dentin matrix protein | PTH | Parathyroid hormone |
DNA | Deoxyribonucleic acid | PTHrP | PTH-related protein |
DPD | Deoxypyridinoline | PTH1R | PTH/PTHrP-1 receptor (PTH/PTHrP-1R) |
DRIP | Vitamin D interacting protein | PYD | Pyridinoline |
DXA | Dual energy x-ray absorptiometry | QCT | Quantitative computed tomography |
ECF | Extracellular fluid | QUS | Quantitative ultrasonography |
EGF | Epidermal growth factor | RANK | Receptor activator of NF-κB |
ER | Estrogen receptor | RANKL | RANK-ligand |
ERK | Extracellular signal-regulated kinase | RDA | Recommended dietary allowance |
FGF | Fibroblast growth factor | RNA | Ribonucleic acid |
FGFR | Fibroblast growth factor receptor | RXR | Retinoid X receptor |
FOS | FBJ osteosarcoma oncogene | SIBLING | Small integrin-binding ligand interacting N-glycoprotein (proteins) |
FRP4 | Frizzled related protein-4 | SOS | Speed of sound |
FRS | FGF receptor substrate | SOX | SRY-Box |
GABA | Gamma (γ)-amino butyric acid | SPP1 | Secreted phosphoprotein 1 (osteopontin) |
GDNF | Glial cell line-derived neurotrophic factor | Src | SRC oncogene |
GDP | Guanosine diphosphate | SRC | Steroid receptor coactivator |
G iα | Alpha subunit of inhibitory GTP-binding protein | STAT | Signal transduction and transcription |
G sα | Alpha subunit of stimulatory GTP-binding protein | SYK | Spleen tyrosine kinase |
G qα | Alpha subunit of a stimulatory GTP-binding protein | TALH | Thick ascending loop of Henle |
GH | Growth hormone | TCF/LEF | T-cell factor/Lymphoid enhancer binding factor |
Gla protein | Matrix gamma carboxyglutamic acid | TGF | Transforming growth factor |
GMP | Guanosine monophosphate | TIO | Tumor induced osteomalacia |
GPCR | G-protein–coupled receptor | TIP | Tuberoinfundibular protein (hypothalamic) |
GRB2 | GRB2-associated binding protein 2 | TNF | Tumor necrosis factor |
GRK | G-protein–coupled receptor kinase | TNSALP | Tissue nonspecific alkaline phosphatase |
GSK3 | Glycogen synthase kinase 3 | TR | Thyroid (hormone) receptor |
GTP | Guanosine triphosphate | TRAF | TNF receptor associated factor |
H + | Hydrogen ion (proton) | TRANCE | TNF-related activation induced cytokine |
HNRPD | Heterogeneous nuclear ribonucleoprotein D | TRAP | Tartrate-resistant acid phosphatase |
HRpQCT | High-resolution peripheral quantitative computed tomography | TREM2 | Triggering receptor expressed on myeloid cells-2 |
HSP | Heat shock protein | TRP | Transient receptor potential (channel) |
VDIR | Vitamin D-interacting repressor | ||
ICTP | Carboxyl-terminal cross-link telopeptide of collagen type I generated by matrix metalloproteinase (see CTX) | VDR | Vitamin D receptor |
IGF | Insulin-like growth factor (somatomedin) | VDRE | Vitamin D response element |
IHH | Indian hedgehog | VEGF | Vascular endothelial growth factor |
IL | Interleukin | WIF | WNT inhibitory factor |
IP 3 | Inositol-1,4,5-trisphosphate | WINAC | WSTF including nucleosome assembly complex |
ITAM | Immunoreceptor tyrosine-based motif | WSTF | Williams syndrome transcription factor |
K + | Potassium | XHR | X-linked hypophosphatemic rickets |
Protein | Gene | Chromosome | OMIM a |
---|---|---|---|
Adaptor-related protein complex 2 | AP2S1 | 19q13.31 | 602242 |
Adenylyl cyclase | ADCY3 | 2p23.3 | 600291 |
Adhesion G-protein–coupled receptor A2 | ADGAR2 | 8p11.23 | 606823 |
Adipocyte-, C1q-, and collagen domain-containing (Adiponectin) | ADIPOQ | 3q27.3 | 605441 |
Aggrecan 1 | AGC1 | 15q26.1 | 155760 |
Alkaline phosphatase | ALPL | 1p36.2 | 171760 |
APC gene | APC | 5q22.2 | 611731 |
β-arrestin 1 | ARRB1 | 11q13.4 | 107940 |
ATPase, Ca(2 +)-transporting, plasma membrane, 1 | ATP2B1 | 12q21.33 | 108731 |
ATPase, Ca(2 +)-transporting, Slow twitch | ATP2A2 | 12q24.11 | 108740 |
Axis inhibitor 1 | AXIN1 | 16p13.3 | 603816 |
β1-Catenin | CTNNB1 | 3p22-p21.3 | 116806 |
Bone morphogenetic protein-1 | BMP1 | 8p21.3 | 112264 |
Bone morphogenetic protein-2 | BMP2 | 20p12 | 112261 |
Bone morphogenetic protein-4 | BMP4 | 14q22.2 | 112262 |
Bone morphogenetic protein-7 | BMP7 | 20q13.31 | 112267 |
BMP Receptor 1A | BMPR1A | 10q22.3 | 601299 |
BMP Receptor 2 | BMPR2 | 2q33 | 600799 |
Bromodomain adjacent to zinc-finger domain, 1B (WSTF) | BAZ1B | 7q11.23 | 605681 |
BSND gene (Barttin) | BSND | 1p32.3 | 606412 |
C-type natriuretic protein | NPPC | 2q24-qter | 600296 |
Calbindin (9K) | S100G | Xp22.2 | 302020 |
Calcitonin | CALCA | 11p15.2 | 114130 |
Calcitonin receptor | CALCR | 7q21.3 | 114131 |
Calcium release-activated calcium modulator 1 | CRACM1 | 12q24 | 610277 |
Calcium sensing receptor | CASR | 3q13.3-q21.1 | 601199 |
Calcium channel, voltage dependent, L-type, alpha 1C subunit | CACNA1C | 12p13.33 | 114205 |
Calmodulin 1 | CALM1 | 14q32.11 | 114180 |
Carbonic anhydrase II | CA2 | 8q21.2 | 611492 |
Cartilage-associated protein | CRTAP | 3p22.3 | 605497 |
Cartilage oligomeric matrix protein | COMP | 19p13.11 | 600310 |
β-Catenin | CTNNB1 | 3p22.1 | 116806 |
Cathepsin K | CTSK | 1q21.3 | 603959 |
Caveolin 1 | CAV1 | 7q31.2 | 601047 |
Chloride channel 5 | CLCN5 | Xp11.22 | 300008 |
Chloride channel 7 | CLCN7 | 16p13.3 | 602727 |
Chloride channel, kidney, B | CLCNKB | 1p36.13 | 602023 |
Claudin 2 | CLDN2 | Xq22.3-23 | 300520 |
Claudin 10 | CLDN10 | 13q32.1 | 617579 |
Claudin 14 | CLDN14 | 21q22.13 | 605608 |
Claudin 16 (Paracellin-1) | CLDN16 | 3q28 | 603959 |
Claudin 19 | CLDN19 | 1p34.2 | 610036 |
Cocaine amphetamine regulated transcript | CART | 5q13.2 | 602606 |
Collagen triple helix repeat-containing protein 1 | CTHRC1 | 8q22.3 | 6100635 |
Collagen type I(α1) | COL1A1 | 17q21.33 | 120150 |
Collagen type I(α2) | COL1A2 | 7q22.1 | 120160 |
Collagen type II(α1) | COL2A1 | 12q13.11 | 120140 |
Collagen type III(α1) | COL3A1 | 2q31 | 120180 |
Collagen type IV(α1) | COL4A1 | 13q34 | 120130 |
Collagen type IX(α1) | COL9A1 | 6q13 | 120210 |
Collagen type X(α1) | COL10A1 | 6q22.1 | 120110 |
Collagen type XI(α1) | COL11A1 | 1p21 | 120280 |
Colony stimulating factor—Macrophage | CSF1 | 1p13.3 | 120420 |
CSF receptor—Macrophage (c-Fms) | CSF1R | 5q32 | 164770 |
Cholesterol 25-hydroxylase | CH25H | 10q23.31 | 604551 |
Core binding factor, beta subunit | CBFB | 16q22.1 | 121360 |
Cyclic AMP-responsive binding protein | CREB1 | 2q33.1 | 123810 |
Cyclic AMP response element-binding protein 3-like 1 | CREB3L1 | 11p11.2 | 616215 |
Cytochrome P450, III A, 4 | CYP3A4 | 7q22.1 | 124010 |
Cytochrome P450, subfamily IIR, polypeptide 1 (25-Hydroxylase) | CYP2R1 | 11p15.2 | 608713 |
Cytochrome P450, subfamily XXVIIB, polypeptide 1 (25OHD-1α hydroxylase) | CYP27B1 | 12q14.1 | 609506 |
Cytochrome P450, family 24, subfamily A, polypeptide 1 (25OHD-24 hydroxylase) | CYP24A1 | 20q13.2 | 126065 |
7-Dehydrocholesterol reductase | DHCR7 | 11q13.4 | 602858 |
Dentin matrix acidic phosphoprotein 1 | DMP1 | 4q22.1 | 600980 |
Dikkopf, xenopus, homolog of, 1 | DKK1 | 10q21.1 | 605189 |
Disheveled 1 | DVL1 | 1p36.33 | 601365 |
Distal-less homeobox 5 | DLX5 | 7q21.3 | 600028 |
Ectonucleotide pyrophosphatase/phosphodiesterase 1 | ENPP1 | 6p23.2 | 173335 |
Elastin | ELN | 7q11.23 | 130160 |
Endogenous retroviral family W, member 1 | ERVW1 | 7q21.2 | 604659 |
Ephrin-B1 | EFNB1 | Xq13.1 | 300035 |
Ephrin-B2 | EFNB2 | 13q33.3 | 600527 |
Ephrin receptor EPHA1 | EPHA1 | 7q34-q35 | 179610 |
Epidermal growth factor | EGF | 4q25 | 131530 |
Family with sequence similarity 20, member A | FAM20A | 17q24.2 | 611062 |
Family with sequence similarity 20, member C | FAM20C | 7p22.3 | 611061 |
Fibroblast growth factor-1 (acidic) | FGF1 | 5q31 | 131220 |
Fibroblast growth factor-2 (basic) | FGF2 | 4q28.1 | 134920 |
Fibroblast growth factor-5 | FGF5 | 4q21 | 165190 |
Fibroblast growth factor-7 | FGF7 | 15q21.2 | 148180 |
Fibroblast growth factor-18 | FGF18 | 5q35.1 | 603726 |
Fibroblast growth factor-21 | FGF21 | 19q33.33 | 609436 |
Fibroblast growth factor-23 | FGF23 | 12p13.32 | 605380 |
Fibroblast growth factor receptor-1 | FGFR1 | 8p11.2-p11.1 | 136350 |
Fibroblast growth factor receptor-2 | FGFR2 | 10q26.13 | 176943 |
Fibroblast growth factor receptor-3 | FGFR3 | 4p16.3 | 134934 |
Fibroblast growth factor receptor-4 | FGFR4 | 5q35 → qter | 134935 |
FK506-binding protein | FKBP10 | 17q21.2 | 607063 |
Four-and-a-half Lim domains | FHL1 | Xq26.3 | 300163 |
Frizzled, drosophila, homolog of | FZD1 | 7q21.13 | 603408 |
Frizzled-related protein-4 | FRP4 | 7p14-p13 | 606570 |
Gamma-carboxyglutamic acid protein, bone (Osteocalcin) | BGLAP | 1q22 | 112260 |
GATA-binding protein 3 | GATA3 | 10p14 | 131320 |
Glial cells missing, Drosophila, homolog of, 2 | GCM2 | 6p24.2 | 603716 |
Glycogen synthase kinase 3-alpha | GSK3A | 19q13.2 | 606784 |
Group-specific component (DBP) | GC | 4q13.3 | 139200 |
Growth hormone 1 | GH1 | 17q23.3 | 139250 |
Growth hormone receptor | GHR | 5p13-p12 | 600946 |
Guanine nucleotide binding protein, alpha stimulating | GNAS1 | 20q13.2 | 139320 |
Guanine nucleotide binding protein, alpha 11 | GNA11 | 19p13.3 | 139313 |
Hairless | HR | 8p21.1 | 602302 |
Hematopoietic transcription factor PU.I | SPI1 | 11p11.2 | 165170 |
Heterogeneous nuclear ribonucleoprotein D | HNRPD | 4q21.1-q21.2 | 601324 |
HNF1 homeobox B | HNF1B | 17q12 | 189907 |
Indian hedgehog | IHH | 2q35 | 600726 |
Inhibitor of kappa light polypeptide gene enhancer … gamma | IKBKG | Xq28 | 300248 |
Inositol trisphosphate receptor | ITPR1 | 3p26.1 | 147265 |
Insulin-like growth factor-I | IGF1 | 12q22-q24.1 | 147440 |
IGF-I receptor | IGF1R | 15q25-q26 | 147370 |
Integrin αv | ITGAV | 2q31 | 193210 |
Integrin β3 | ITGB3 | 17q21.32 | 173470 |
Integrin-binding sialoprotein | IBSP | 4q22.1 | 147563 |
Interferon-induced transmembrane protein family 5 | IFITM5 | 11p15.5 | 614757 |
Interleukins-1-beta | IL1B | 2q14.1 | 147720 |
Interleukin-6 | IL6 | 7p15.3 | 147620 |
Interleukin 6-dependent DNA binding protein | CEBPB | 20q13.13 | 189965 |
Klotho | KL | 13q13.1 | 604824 |
Leptin | LEP | 7q31.3 | 164160 |
Lipocalin 2 | LCN2 | 9q34.11 | 600181 |
Low-density lipoprotein receptor-related protein 2 (Megalin) | LRP2 | 2q31.1 | 600073 |
Low-density lipoprotein receptor-related protein 5 | LRP5 | 11q13.2 | 603506 |
Low-density lipoprotein receptor-related protein 6 | LRP6 | 12p13.2 | 603507 |
V-Mak musculoaponeurotic fibrosarcoma oncogene family, protein B | MAFB | 20q12 | 608968 |
Matrilin 3 | MATN3 | 2p24.1 | 602109 |
Matrix extracellular phosphoglycoprotein | MEPE | 4q21.1 | 605912 |
Matrix gamma carboxyglutamic acid (Gla protein) | MGP | 12p12.3 | 154870 |
Matrix metalloproteinase 9 | MMP9 | 20q13.12 | 120361 |
Mediator complex subunit 4 (vitamin D interacting protein) | MED4 | 13q14.2 | 605718 |
Membrane-bound transcription factor protease site 2 | MBTPS2 | Xp22.12 | 300294 |
Methyl-CpG-binding domain protein 4 | MBD4 | 3q21.3 | 603574 |
Mitochondrial RNA-processing endoribonuclease, RNA component of | RMRP | 9p13.3 | 157660 |
Mothers against decapentaplegic, drosophila, homolog of, 2 | SMAD2 | 18q21.1 | 601366 |
Natriuretic peptide precursor C | NPPC | 2q37.1 | 600296 |
Natriuretic peptide receptor B | NPR2 | 9p13.3 | 108961 |
Natriuretic peptide receptor C | NPR3 | 5p13.3 | 108962 |
Noggin, mouse, homolog of | NOG | 17q22 | 602991 |
Notch, drosophila, homolog of 1 | NOTCH1 | 9q34.3 | 190198 |
Nuclear factor-κB, Subunit 1 | NFKB1 | 4q24 | 164011 |
Nuclear factor-κB, Inhibitor (IκB) | NFKB1A | 14q13.2 | 164008 |
Nuclear factor of activated T cells, cytoplasmic, calcineurin-dependent 1 | NFATC1 | 18q23 | 600489 |
ORAI calcium release-activated calcium modulator 1 | ORAI1 | 12q24.31 | 610277 |
Osteoclast-associated receptor | OSCAR | 19q13.42 | 606862 |
Osteocrin | OSTN | 3q28 | 610280 |
Osteopetrosis-associated transmembrane protein 1 | OSTM1 | 6q21 | 607649 |
Paired box gene 1 | PAX1 | 20p11.22 | 167411 |
Parathyroid hormone | PTH | 11p15.2 | 168450 |
PTH-related/like protein | PTHLH | 12p11.22 | 168470 |
PTH 1 receptor | PTH1R | 3p21.31 | 168468 |
PTH 2 receptor | PTH2R | 2q34 | 601469 |
Patched 1 | PTCH1 | 9q22.3 | 601309 |
Peptidyl-prolyl isomerase B (CypB) | PPIB | 15q22.31 | 123841 |
Peroxisome proliferator-activated receptor gamma | PPARG | 3p25.2 | 601487 |
Phosphate-regulating gene with homologies to endopeptidases on the X chromosome | PHEX | Xp22.1 | 300550 |
Phosphodiesterase 3A, cGMP-inhibited | PDE3A | 12p12.2 | 123805 |
Phosphodiesterase 4D, cAMP-specific | PDE4D | 5q11.2-q12.1 | 600129 |
Phospholipase Cβ-1 | PLCB1 | 20p12.3 | 607120 |
Plastin 3 | PLS3 | Xq23 | 300131 |
Pleckstrin homology domain containing protein, family M, member 1 | PLEKHM1 | 17q21.31 | 611466 |
Potassium channel, inwardly rectifying, subfamily 1, member 1 | KCNJ1 | 11q24.3 | 600359 |
Potassium channel, inwardly rectifying, subfamily J, member 10 | KCNJ10 | 1q23.2 | 602208 |
Potassium channel, voltage-gated, Shaker- related subfamily, member 1 | KCNA1 | 12p13.32 | 176260 |
Pregnane X receptor | NR112 | 3q13-q21 | 603065 |
Procollagen-lysine,2-oxoglutarate 5-dioxygenase 1 | PLOD1 | 1p36.22 | 153454 |
Procollagen-lysine,2-oxoglutarate 5-dioxygenase 2 | PLOD2 | 3q24 | 601865 |
Prolyl 3-hydroxylase 1 | P3H1 | 1p34.2 | 610339 |
Protein disulfide isomerase, family A, member 3 (MARRS-BP) | PDIA3 | 15q14.3 | 602406 |
Protein kinase A | PRKAR1A | 17q24.3 | 188830 |
Protein phosphatase 1, regulatory subunit 1B | PPP1R1B | 17q12 | 604399 |
Protein phosphatase 3, catalytic subunit, alpha isoform (calcineurin A) | PPP3CA | 4q24 | 114105 |
Receptor activator of NF-κB (RANK) | TNFRSF11A | 18q22.1 | 603499 |
RANK-Ligand (RANKL) | TNFSF11 | 13q14.11 | 602642 |
Retinoid X receptor α | RXRA | 9q34.3 | 180245 |
Reversion-inducing cysteine-rich protein with kazal motifs | RECK | 9p13.3 | 605227 |
Runt-related transcription factor 2 | RUNX2 | 6p21.1 | 600211 |
Sclerostin | SOST | 17q21.31 | 605740 |
Secreted frizzled-related protein 1 | SFRP1 | 8p11.21 | 604156 |
Secreted phosphoprotein 1(Osteopontin) | SPP1 | 4q22.1 | 166490 |
Secreted protein, acidic, cysteine-rich (Osteonectin) | SPARC | 5q33.1 | 182120 |
Serpin peptidase inhibitor, clade H, member 1 (Heat shock protein 47) | SERPINH1 | 11q13.5 | 600943 |
Sirtuin 1 | SIRT1 | 10q21.3 | 604479 |
Smoothened | SMOH | 7q31-q32 | 601500 |
Sodium phosphate cotransporter Solute carrier family 34, member 1 (NPT2a/NaPi-IIa) | SLC34A1 | 5q35.3 | 182309 |
Sodium phosphate cotransporter Solute carrier family 34, member 2 (NPT2b/NaPi-IIb) | SLC34A2 | 4p15.2 | 604217 |
Sodium phosphate cotransporter Solute carrier family 34, member 3 (NPT2c/Na-Pi-IIc) | SLC34A3 | 9q34.3 | 609826 |
Solute carrier family 4 (anion exchanger), member 2 | SLC4A2 | 7q36.1 | 109280 |
Solute carrier family 8 (sodium-calcium exchanger), member 1 – cardiac | SLC8A1 | 2p22.1 | 182305 |
Solute carrier family 8 (sodium-calcium exchanger), member 2 – skeletal | SLC8A2 | 19q13.32 | 601901 |
Solute carrier family 9, member 3 | SLC9A3 | 5p15.3 | 182307 |
Solute carrier family 9, member 3, regulator 1 (NHERF1) | SLC9A3R1 | 17q25.1 | 604990 |
Solute carrier family 12 (sodium/potassium/chloride transporter), member 1 | SLC12A1 | 15q21.1 | 600839 |
Solute carrier family 12 (sodium/chloride transporter), member 3 | SLC12A3 | 16q13 | 600968 |
Solute carrier family 20 (phosphate transporter), member 2 | SLC20A2 | 8p11.21 | 158378 |
Sonic hedgehog | SHH | 7q36.3 | 600725 |
Sorting nexin 10 | SNX10 | 7p15.2 | 614780 |
Sphingosine-1-phosphate | S1PR1 | 1p21.2 | 601974 |
SRY-box 9 | SOX9 | 17q24.3 | 608160 |
Stromal interaction molecule 1 | STIM1 | 11p15.4 | 605921 |
T-Box 1 | TBX1 | 22q11.2 | 602054 |
T-cell factor/lymphoid enhancer binding factor | LEF1 | 4q25 | 153245 |
T-cell immune regulator 1 | TCIRG1 | 11q13.2 | 604592 |
Tartrate-resistant acid phosphatase | ACP5 | 19p13.2 | 171640 |
Transcription factor 3/VDR interacting repressor | TCF3 | 19p13.3 | 147141 |
Transcription factor Sp7 (Osterix) | SP7 | 12q13.13 | 606633 |
Transforming growth factor, Beta 1 | TGFB1 | 19q13.2 | 190180 |
Transient receptor potential cation channel, subfamily M, member 6 | TRPM6 | 9q21.13 | 607009 |
Transient receptor potential cation channel, subfamily M, member 7 | TRPM7 | 15q21.2 | 605692 |
Transient receptor potential cation channel, subfamily V, member 4 | TRPV4 | 12q24.1 | 605427 |
Transient receptor potential cation channel, subfamily V, member 5 | TRPV5 | 7q34 | 606679 |
Transient receptor potential cation channel, subfamily V, member 6 | TRPV6 | 7q34 | 606680 |
Transmembrane protein 38B | TMEM38B | 9q31.2 | 611236 |
Triggering receptor expressed on myeloid cells 2 | TREM2 | 6p21.1 | 605086 |
Tumor necrosis factor-α | TNF | 6p21.33 | 191160 |
Tumor necrosis factor ligand superfamily, member 11 (OPGL, RANKL, TRANCE) | TNFSF11 | 13q14.11 | 602642 |
Tumor necrosis factor receptor superfamily, Member 11A | TNFRSF11A | 18q21.33 | 603499 |
Tumor necrosis factor receptor superfamily, member 11B (osteoprotegerin) | TNFRSF11B | 8q24.12 | 602643 |
Tumor necrosis factor-associated factor 6 | TRAF6 | 11p12 | 602355 |
Tuberoinfundular peptide 39 | TIP39 | 19q13.33 | 608386 |
UDP-N-acetyl-alpha-D-galactosamine: Polypeptide N-acetylgalactosaminyl-transferase 3 | GALNT3 | 2q24.3 | 601756 |
V-Fos FBJ murine osteosarcoma | FOS | 14q24.3 | 164810 |
Vascular endothelial growth factor | VEGF | 6p12 | 192240 |
Vitamin D binding protein | GC | 4q13.3 | 139200 |
Vitamin D receptor | VDR | 12q12-q14 | 601769 |
Voltage-gated calcium channel α 1 | CACNA1C | 12p13.3 | 114205 |
Wingless-type mouse mammary tumor virus (MMTV) integration site, member 1 | WNT1 | 12q13.12 | 164820 |
Wingless-type mouse mammary tumor virus (MMTV) integration site, member 9 | WNT9A | 1q42 | 602863 |
WNT inhibitory factor 1 | WIF1 | 12q14.3 | 605186 |
Xenotropic and polytropic retrovirus receptor | XPR1 | 1q25.3 | 605237 |
a Online Mendelian Inheritance in Man – https://www.omim.org/
Ca 2 + also enters the cytosol through the plasma membrane store-operated Ca 2 + channel—ORAI1 (encoded by ORAI1 ). Store-operated Ca 2 + channels are active in cells that are electrically nonexcitable, such as lymphocytes and other immune cells. Both voltage-gated and store-operated Ca 2 + channels often coexist in the same cell. The type of Ca 2 + channel used in a specific cell is determined by the expression of STIM1 (see Table 9.1 ) encoding stromal interaction molecule 1—a protein that transverses the membrane of the endoplasmic reticulum. When the Ca 2 + content (e.g., “store”) of the endoplasmic reticulum is depleted, STIM1 unfolds and bridges the cytosolic distance between the endoplasmic reticulum and the cell’s plasma membrane where it binds to and opens the ORAI1 channel, thereby permitting Ca 2 + influx into the cytoplasm and the restoration of Ca 2 + stores within cytoplasmic organelles. At the same time that it activates the ORAI1 Ca 2 + channel, STIM1 inhibits the voltage-gated Ca 2 + channel. Loss-of-function mutations in ORAI1 have been identified in patients with severe combined immune deficiency and tubular myopathy.
In addition to traversing rapidly across the cell’s plasma membrane through voltage-gated and store-operated channels, Ca 2 + enters the cytosol, but at a markedly slower rate, through bifunctional membrane-associated IP 3 receptors that also serve as calcium channels. Encoded by ITPR1 , the tetrameric IP 3 receptor has six transmembrane domains and a pore-forming loop between the fifth and sixth transmembrane segments. Whereas many IP 3 receptor/channels are expressed in the membranes of the endoplasmic reticulum where IP 3 activation leads to depletion of Ca 2 + stored in the endoplasmic reticulum, thereby triggering store-operated calcium channel activation, only one to two such channels are expressed in the plasma membrane of each cell. Ca 2 + is translocated not only through specific Ca 2 + channels but also through paracellular transport channels. Ca 2 + is extruded from cell cytoplasm by calcium and energy-dependent adenosine triphosphate (ATP)-driven calcium pumps and in exchange for sodium (Na + ) through H + /ATPase and Na + /Ca 2 + exchangers. The Ca 2 + -ATPases are encoded by four genes: ATP2B1 encodes a 1220-amino-acid (AA) membrane protein with a cyclic adenosine monophosphate (AMP)-dependent protein kinase domain and a carboxyl domain to which a calbindin (a calcium binding protein) attaches that links hydrolysis of ATP with transport of cations across membranes. The Ca 2 + -ATPases have the capacity to transport Ca 2 + against high concentration gradients. The Na + /Ca 2 + exchangers are widely distributed plasma membrane Ca 2 + export proteins. A homolog of the human Na + /Ca 2 + exchanger is comprised of 10 transmembrane domains consisting of two sets of five transmembrane helices in inverted reverse order, with the amino and carboxyl termini within the cytoplasm. Transmembrane helices 2, 3, 7, and 8 form an ion-binding pocket with three low-affinity Na + and one high-affinity Ca 2 + binding sites symmetrically arranged in a diamond formation. Through conformational changes of the structure of the Na + /Ca 2 + exchanger, the Na + and Ca 2 + binding sites are alternately exposed to either the intracellular or extracellular surfaces of the cell’s plasma membrane. As Na + from the extracellular space occupies its three binding sites, the binding affinity for Ca 2 + decreases, thereby releasing this cation into the cytosol and reversing the orientation of the Na + /Ca 2 + channel; inasmuch as cytosolic levels of Na + are low, these cations are then released into the cytosol, restoring the affinity of the exchanger for Ca 2 + . The human cardiac Na + /Ca 2 + exchanger is encoded by SLC8A1 and the human skeletal muscle Na + /Ca 2 + exchanger by SLC8A2 . Within the cell, cytosolic Ca 2 + is transported across the membrane and into the endoplasmic reticula of muscle cells through Ca 2 + -ATPase channels encoded by ATP2A2 ; linkage of small ubiquitin-like modifier type 1 (sumoylation) to this Ca 2 + -ATPase extends its half-life and increases its intrinsic activity.
The circulating concentration of Ca 2 + is detected by the CaSR, encoded by CASR , a G-protein–coupled transmembrane heptahelical receptor (GPCR) expressed on the plasma membrane of the PTH-secreting chief cells of the parathyroid glands, epithelial cells of the renal tubules, osteoblasts, and other tissues. When the ambient Ca 2 + concentration declines, CaSR signaling in the parathyroid glands through Gq 11α (encoded by GNA11 ) activates the intracellular phosphatidylinositol 4,5 bisphosphate signal transduction pathway, leading to rapid release of Ca 2 + from intracellular storage sites and subsequent increase in synthesis and secretion of PTH. In turn, PTH (and PTHrP) may act through binding to its stimulatory GPCR (encoded by PTH1R ) present on the plasma membranes of osteoblasts to enhance expression of TNFSF11 encoding the receptor activator of nuclear factor kappa-B (NF-κB) ligand (RANK-ligand) thereby stimulating osteoclastogenesis and increasing the rate of bone resorption, thus releasing calcium and increasing its extracellular concentration. PTH also acts on epithelial cells lining the distal renal tubule to enhance renal reabsorption of filtered Ca 2 + and synthesis of calcitriol (thereby increasing intestinal absorption of Ca 2 + ) while suppressing proximal renal tubular reabsorption of filtered phosphate directly and indirectly by enhancing osteocytic synthesis of fibroblast growth factor-23 (FGF23). Increased serum concentrations of Ca 2 + reciprocally suppress CaSR activity and PTH synthesis. Intracellular signaling induced by PTH proceeds primarily through the stimulatory guanosine phosphate-binding protein composed of alpha (G sα ), beta, and gamma subunits. After substitution of GTP for guanosine diphosphate (GDP) on the α subunit, G sα dissociates from its linked βϐ subunits. G sα then stimulates intracellular adenylyl cyclase (encoded by ADCY3 ) that converts ATP to cyclic AMP and releases it from the cytoplasmic surface of the plasma membrane of the PTH-responsive cell. Cyclic AMP in turn binds to the regulatory alpha subunit of protein kinase A (encoded by PRKAR1A ), thereby initiating intracellular signaling. The catalytic subunits of protein kinase A phosphorylate a number of intracellular proteins, including the cyclic AMP response binding protein (encoded by CREB1 ) that in turn initiates transcription of cyclic AMP target genes. CREB1 is deactivated by one of several phosphodiesterases, including those encoded by PDE4D and PDE3A . By increasing activity of 25-hydroxyvitamin D-1α hydroxylase, PTH stimulates renal synthesis of calcitriol, the active metabolite of vitamin D; calcitriol increases calcium absorption in the proximal small intestinal tract and distal renal tubule, phosphate absorption in the intestine and kidney, and calcium mobilization from bone stores by enhancing osteoclastogenesis; calcitriol downregulates the synthesis and secretion of PTH (see Fig. 9.1 ).
In the gastrointestinal tract, calcium is absorbed by both active transcellular and passive paracellular processes. During growth in younger subjects and when calcium intake and consequently intraintestinal calcium concentrations are low, active transcellular absorption of calcium predominates; when calcium intake is adequate or high, passive paracellular absorption of calcium in the proximal intestine predominates. Transcellular gastrointestinal absorption of calcium is an active and saturable process that is primarily stimulated by the active metabolite of vitamin D 3 (calcitriol) by regulating the availability of transmembrane calcium “pumps” and channels on the luminal/apical and basolateral surface membranes of duodenal enterocytes. Calcitriol acting through the vitamin D receptor (VDR) increases duodenal expression of calcium transporter protein-1 (encoded by transient receptor potential cation channel 6 [ TRPV6 ])—a luminal calcium channel with six transmembrane domains and intracytoplasmic amino and carboxyl terminals and a pore region between the fifth and sixth transmembrane domains that forms a homotetramer or heterotetramer combined with TRPV5; TRPV5 and TRPV6 specifically permit Ca 2 + permeation at physiological levels of this cation. Calcium is also actively absorbed in the intestinal tract by mechanisms that are independent of the VDR. Thus the expression of TRPV6 is increased by both estrogen and prolactin; prolactin increases transcellular absorption of calcium independently of calcitriol. After entering the cytoplasm of the enterocyte, Ca 2 + travels from its apical to its basolateral membrane either within a lysosomal vesical bound to vitamin D-dependent calbindin D 9k (encoded by S100G ), a protein with two high-affinity calcium binding sites, or within the cytoplasm linked to calmodulin (encoded by CALM1 ). Calmodulin 1 is a 149 AA protein with an amino-terminal “lobe” linked to a carboxyl-terminal “lobe” that can assume more than 30 three-dimensional conformations. Each lobe has two globular calcium binding domains consisting of two helix-loop-helix motifs connected by an α-helical linker; calcium binding exposes hydrophobic “pockets” that allow calmodulin to bind to and regulate the activity of target proteins. When bound to a target protein, calmodulin can assume an exceedingly large number of conformations. This versatility enables calmodulin to act as a calcium sensor for many different proteins subserving distinct processes within a single cell, including voltage-gated calcium channels and calcium/calmodulin-dependent protein kinases. At the basolateral membrane, Ca 2 + dissociates from calbindin and calmodulin and is then extruded into the extracellular space across the basolateral membrane through a basolateral Ca 2 + -Mg 2 + -dependent ATPase calcium channel (encoded by ATP2B1 ). When calcium intake is adequate or high, the bulk of ingested calcium is passively absorbed through paracellular channels between enterocytes in the jejunum and ileum. Calcitriol regulates synthesis of several tight junction proteins, including claudins-2 and -12, cadherin-17, and aquaporin 8.
Intestinal calcium absorption is influenced by vitamin D status, its food source (the bioavailability of calcium in cow milk formulas is 38%, that in human breast milk is 58%); the form of the calcium salt in dietary supplements; and the presence in food of inhibitors of calcium absorption, such as phytates, oxalates, or phosphates (e.g., cola beverages). Intestinal calcium absorption is increased during adolescence, pregnancy, and lactation, and depressed in patients with nutritional or functional vitamin D deficiency, chronic renal disease, and hypoparathyroidism. The amount of calcium ingested influences the net amount of calcium absorbed; the lower the calcium intake, the greater is the efficiency of its absorption. In the adult, when the dietary calcium intake is less than 200 mg/d, fecal calcium excretion exceeds intake; thus active absorption of calcium cannot compensate for very low intake. As dietary calcium increases from 200 to 1000 mg/d, active calcium absorption increases but at a progressively decreasing rate. When dietary calcium exceeds 1000 mg/d, active calcium absorption remains relatively constant at 400 mg/d, but passive absorption of calcium through paracellular channels continues to increase. Thus hypercalcemia may result from dietary calcium excess as in the milk-alkali syndrome. PTH indirectly increases intestinal calcium absorption by enhancing renal 25-hydroxyvitamin D-1α hydroxylase activity and hence calcitriol synthesis. Growth hormone (GH) and estrogens also increase intestinal absorption of calcium; glucocorticoids and thyroid hormone inhibit this process. Calcium is excreted into the intestinal tract in the ileum and in pancreatic and biliary secretions.
Low calcium intake is associated with increased fracture rate in children and adolescents, and therefore adequate dietary intake of calcium during infancy, childhood, and adolescence is necessary to attain a peak bone mass that may lessen the risk of fracture and the development of osteoporosis. To ensure optimal mineralization of the developing skeleton, age-related dietary intakes of elemental calcium for infants, children, and adolescents have been recommended ( Table 9.2 ). As a nutrient essential for bone mineralization, intake of calcium is optimal when skeletal calcium content is maximal for the age and gender of the subject. In normally weighted adolescents, a calcium intake of or above 1600 mg per day results in maximal calcium retention (approximately 35%). Calcium retention and bone mass increase as body mass index (BMI) rises when calcium intake is sufficient. The retention of oral calcium is dependent not only on the level of nutrient intake but also upon gender and race: white adolescent males retain more calcium than do white girls (37% vs. 30%); black adolescent girls retain more calcium than do white females (38% vs. 30%). Besides dietary intake of calcium and vitamin D status, the most significant modifiable determinant of bone mineralization is weight-bearing physical activity.
Age (y) | Calcium (mg/day) | Calcium (Upper Limit) | Vitamin D (IU/day) | Minimum [250OHD] (ng.mL) |
---|---|---|---|---|
< 0.5 | 200 | 1000 | 400 | 20 |
0.5–1 | 260 | 1500 | 400 | 20 |
1–3 | 700 | 2500 | 600 | 20 |
4–8 | 1000 | 2500 | 600 | 20 |
9–13 | 1300 | 3000 | 600 | 20 |
14–18 | 1300 | 3000 | 600 | 20 |
Pregnant or lactating | 1300 | 3000 | 600 | 20 |
Calcium is excreted primarily by the kidney. After the ultrafiltrable portion (ionized, complexed, chelated) of plasma calcium crosses the renal glomerular basement membrane, more than 98% of filtered calcium is reabsorbed—primarily in the proximal convoluted tubule (60%) but also in the thick ascending limb of the loop of Henle (TALH) (20%), distal convoluted tubule (10%), connecting tubule (3%), and collecting duct (10%). In the proximal convoluted tubule, the bulk of calcium is passively reabsorbed through paracellular channels; a small amount of calcium is reabsorbed actively, a process regulated by both PTH and calcitonin. In the cortical TALH, resorption of filtered calcium is regulated in part through the influence of Na + -K + -2Cl − ion channels and the CaSR upon the permeability of paracellular channels to calcium. Active transcellular movement of calcium in the TALH is stimulated by PTH and calcitonin. In the distal convoluted renal tubule, active transcellular reabsorption of 5% to 10% of filtered calcium occurs through a calcium channel encoded by TRPV5 that is expressed on the apical membrane of renal tubular epithelial cells, a process regulated by calcitriol and PTH. In the cytoplasm, calbindin D 9k then shuttles Ca 2 + across the cell for extrusion by ATP2B1 channels and the Na + -Ca 2 + exchanger type 1 (encoded by SLC8A1 ) on the basolateral membrane of the renal tubular cell. Calcitriol, PTH, low dietary calcium, estrogens and androgens, and acid/base balance increase expression of TRPV5 and, thus renal tubular reabsorption of calcium. PTH also enhances posttranslational phosphorylation of TRPV5 and hence its movement to and insertion into the apical membrane of the renal tubular cell and inhibits its endocytosis, the paired effects thus resulting in increased numbers of TRPV5 channels on the apical surface of the renal tubular cell. FGF23 and αklotho (encoded by KL ), factors essential for normal phosphate homeostasis, increase the intracellular retention and hence decrease the abundance and hence the activity of the TRPV5 calcium channel on the apical surface of the renal tubular cell. Calmodulin, an intracellular Ca 2 + -binding protein that also binds to and inhibits the action of TRPV5, modulates renal tubular reabsorption of Ca 2 + , a process reversed by PTH-mediated phosphorylation of the TRPV5 calbindin-binding site.
In the TALH, calcium and magnesium are reabsorbed through voltage-driven paracellular channels (in part through paracellin-1, a tight junction protein channel). Paracellular transport of solute involves passive movement of ions along channels in the tight junctions between adjacent cells that are in complete contact with one another. Claudins are proteins present in the plasma membranes of the adjacent cells of the TALH that have formed tight junctions; claudins have four extracellular domains that associate with one another and selectively influence paracellular solute permeability and movement. Claudins-14, -16, and -19 (encoded by CLDN14 , – 16, -19 , respectively) are expressed in the tight junctions of the cells lining the TALH; the expression of CLDN14 is partially regulated by the CaSR. Cells of the TALH also express CASR on their basolateral membrane; when activated by peritubular Ca 2 + , the CaSR decreases renal tubular reabsorption of calcium through the paracellular channels by inhibiting activity of the Na + -K + -2Cl − transporter and lowering lumen-positive voltage. Increased glomerular filtration and/or decreased renal tubular reabsorption increase renal excretion of calcium, phosphate, and magnesium. Urinary excretion of calcium is augmented by increased dietary intake, hypercalcemia of diverse pathophysiology (with the exception of that associated with familial hypocalcemic hypercalciuria), expansion of extracellular volume, metabolic acidosis, and loop diuretics (e.g., furosemide). PTH and PTHrP increase renal tubular reabsorption of Ca 2 + , whereas glucocorticoids, mineralocorticoids, and Ca 2 + itself suppress Ca 2 + reabsorption (see Fig. 9.1 ).
Plasma Ca 2 + concentrations are monitored by the CaSR, a 1078 AA, seven transmembrane GPCR encoded by CASR whose extracellular domain recognizes and binds Ca 2 + , Mg 2 + , gadolinium, aromatic AA, antibiotics, and other compounds. The CaSR is expressed on the cell’s plasma membrane as a homodimer linked by disulfide bonds between extracellular domain cysteine residues 129 and 131 of each CaSR; its extracellular domain of 612 AA binds Ca 2 + . Homodimerization of the CaSR takes place in the endoplasmic reticulum after core N-linked glycosylation; homodimeric CaSR is then packaged within the Golgi apparatus where it is further glycosylated and transported to the cell membrane. The amino-terminal extracellular domain of the CaSR is composed of 612 AA with five Ca 2 + binding sites constructed in a “Venus flytrap” configuration; there are 250 AA in the transmembrane domain composed of seven transmembrane helices and three alternating extracellular and intracellular loops, and 216 AA in the carboxyl-terminal intracellular domain. The second extracellular loop contains sites (Asp758, Glu759, Glu767) that modulate the “sensitivity” of the CaSR to Ca 2 + . Within the intracellular domain of the CaSR are three protein kinase C phosphorylation sites; phosphorylation of Thr888 suppresses Ca 2 + -mediated CaSR intracellular signal transduction. Through its binding of Ca 2 + , the CaSR finely regulates the extracellular concentration of this cation by modulating the secretion of PTH and the renal tubular reabsorption of filtered calcium. The CaSR is a member of the C family of GPCRs with extremely long extracellular domains (500–600 AA residues) and homologies with receptors that bind glutamate, γ-amino butyric acid (GABA), and pheromones, as well as with the taste receptors. The very long extracellular domain is extensively glycosylated, a posttranslational modification essential for efficient movement of the receptor to the cell surface. The CaSR is located on the plasma membrane of parathyroid chief cells, at the apical or basolateral membranes of most renal tubular cells—particularly those in the TALH and the collecting ducts, on the cell membranes of the calcitonin-synthesizing parafollicular C cells of the thyroid gland, in cartilage and bone, lungs, adrenal glands, breast, intestines, skin, lens, placental cytotrophoblasts, and nervous tissue. Expression of CASR is regulated in part by glial cell missing homolog 2 ( GCM2 ), encoding a transcription factor essential for formation of the parathyroid glands, and enhanced by calcitriol, high Ca 2 + levels, and interleukin-1β. Insertion of the CaSR into the cell’s plasma membrane is mediated by an agonist-driven insertional pathway antagonized by the cooperative interaction of β-arrestin 1 (encoded by ARRB1 ) and an adaptor-related protein complex 2 ( AP2S1 ), the latter complex facilitating retrograde movement (endocytosis) of the CaSR from the cell surface and its return to the cytoplasm.
The intracellular signal of the CaSR is transduced by the G αq and G α/11 ( GNA11 ) family of GPCRs that activates PLCβ-1 (encoded by PLCB1 ), that then cleaves phosphatidylinositol 4,5-bisphosphate to diacylglycerol (DAG) and IP 3 . In the parathyroid gland, IP 3 mobilizes stored intracellular calcium, whereas DAG activates the mitogen-activating protein kinase (MAPK) signal transduction pathway that leads to decrease in PTH secretion. Activation of the CaSR also stimulates activity of phospholipases A 2 and D and PKB (AKT) and PKC but inhibits that of adenylyl cyclase, the latter through stimulation of adenylate cyclase-inhibitory Gα i activity. Increases in plasma Ca 2 + and rising cytosolic levels of Ca 2 + within the parathyroid chief cells suppress expression of PTH and increase degradation of PTH messenger ribonucleic acid (mRNA), thereby limiting synthesis and secretion of PTH; CaSR signaling also inhibits proliferation of chief cells. Decline in Ca 2 + leads to decrease in CaSR activity, lower intracellular Ca 2 + values, and thence to increased PTH secretion, thus enabling the CaSR to exercise minute-to-minute control over the release of PTH and hence of the plasma concentration of Ca 2 + .
The serum Ca 2 + concentration is related to polymorphic variants of the CaSR; 70% of individuals are homozygous for alanine at AA 986 within the intracellular domain, 3% are homozygous for serine, and the remainder are heterozygous for the two amino acids. In heterozygous Arg986Ser and homozygous Ser986 subjects, Ca 2 + levels are significantly higher than in those who are homozygous for Ala986. The CaSR also serves as a Mg 2 + sensor and modulates renal tubular reabsorption of this cation decreasing its reabsorption when Mg 2 + levels rise. By binding to the CaSR, aromatic l -amino acids appear to “sensitize” the receptor to a given level of Ca 2 + .
In the kidney, CaSRs are present on the apical (luminal) brush-border membranes of cells in the proximal renal tubule where they are exposed to intratubular Ca 2 + , on basolateral cell membranes in cells in the TALH, and on the apical surface of cells in the distal renal collecting ducts. Functioning through binding to local CaSRs, increasing peritubular concentrations of Ca 2 + and Mg 2 + inhibit renal tubular reabsorption of filtered Ca 2 + and Mg 2 + , respectively. In the kidney, binding of Ca 2 + to the CaSR decreases not only transcellular transport of filtered Ca 2 + but also its paracellular transport in the TALH. Inactivating mutations in CASR result in familial hypocalciuric hypercalcemia, whereas gain-of-function mutations in CASR are associated with autosomal dominant hypoparathyroidism (“familial hypercalciuric hypocalcemia”). Stimulation of the CaSR suppresses PTH-mediated renal tubular Ca 2 + reabsorption. In addition to effects on renal tubular cation transport, the Ca 2 + /CaSR complex inhibits antidiuretic hormone–induced renal tubular permeability to water by decreasing the number of apical aquaporin-2 water channels in the inner medullary collecting ducts, thus leading to polyuria. Stimulation of the CaSR expressed in cells of the renal distal collecting ducts also increases local H + -ATPase activity, resulting in urine acidification.
Acting through the CaSR, increasing serum Ca 2 + concentrations stimulate release of calcitonin from thyroid C cells. The CaSR is expressed throughout the intestinal tract, where it may modulate the changes in intestinal motility that accompany low (increased) and high (depressed) serum Ca 2 + values. In addition, in gastric cells, stimulation of CaSR activity increases release of gastrin into the circulation and thereby intragastric acidity. Expression of the CaSR in the brain suggests a mechanism whereby Ca 2 + may influence neural function locally by modulating neurotransmitter and neuroreceptor function. The CaSR is present on cell membranes of osteoblasts, osteocytes, and osteoclasts, and agonist (Ca 2 + , neomycin, gadolinium) activation of the CaSR stimulates intracellular signal transduction in these cells. Increase in CaSR activity in osteoblasts enhances their proliferation. The CaSR may mediate recruitment of osteoblast precursor cells to sites of high Ca 2 + levels, the residue of local osteoclast activity, one of the factors linking the bone remodeling processes of resorption and formation.
Calcimimetics are agonist drugs that activate the CaSR; calcilytics are antagonists of the CaSR. The CaSR binds and responds not only to Ca 2 + but also to Mg 2 + , selected L-amino acids, and some antibiotics; the latter are designated type I calcimimetics. Synthetic compounds (e.g., phenylalkylamines) that bind to the second and third extracellular loops of the transmembrane domain of the CaSR and allosterically modulate (increase or decrease) the sensitivity of the CaSR to ambient Ca 2 + are designated type II calcimimetic agonists or calcilytic antagonists, respectively. A widely used calcimimetic is cinacalcet [N-[1-(R)-(-)-(1-naphthyl)ethyl]-3-[3-(trifluoromethyl)phenyl]-1-aminopropane hydrochloride]; this agent has been effective in decreasing secretion of PTH in patients with either primary or secondary hyperparathyroidism. Calcilytics decrease the sensitivity of the CaSR to Ca 2 + and thus increase the secretion of PTH and depress renal tubular reabsorption of Ca 2 + . Variants of CASR may prevent its normal biosynthesis (class I), disturb movement of the CaSR protein to the plasma membrane (class II), interfere with binding of ligand to the CaSR (class III), impair activation of the CaSR (class IV), or disrupt receptor function by a currently unknown mechanism (class V).
Phosphate
Second to calcium in abundance, phosphorus is present in humans in organic forms associated with fats, sugars, and proteins and in inorganic forms complexed to sodium, calcium, or magnesium. It is an essential component of deoxyribonucleic acid (DNA) and RNA nucleotides, critical for generation, storage, and utilization of energy as ATP, and irreplaceable as a part of cell membranes, signal transduction pathways, and bone mineral. Serum concentrations of phosphate reflect the balance between the amount of this anion ingested into and absorbed by the duodenum and jejunum of the gastrointestinal tract; the quantity filtered through the renal glomerulus and subsequently reabsorbed by the proximal renal tubule or excreted in urine; its movement between intracellular and extracellular spaces; and the intraosseous interactive dynamics of calcium, phosphate, and the formation and resorption of hydroxyapatite.
Some 85% of body phosphate is deposited in bone as hydroxyapatite. Some 14% of phosphate is intracellular (in the cytosol or mitochondria in the form of inorganic phosphate esters or salts, cell and organelle membrane phospholipids, and phosphorylated metabolic intermediate compounds involved in energy metabolism and formation of ATP and in signal transduction and in the cytosol and nucleus as an essential component of RNA and DNA) or in interstitial fluid or serum (approximately 1%). In serum, phosphate circulates as free orthophosphate anions HPO 4 2− and H 2 PO 4 − (55%), bound to proteins (10%), or complexed to calcium, magnesium, or sodium (35%). At pH 7.4, serum HPO 4 2− and H 2 PO 4 − are present in a molar ratio of 4:1; in alkalotic states the ratio increases, with acidosis it declines (at pH 7.4, 1 mmol/L of orthophosphate = 1.8 mEq/L = 3.1 mg/dL). The serum concentrations of calcium and phosphate are reciprocally related under normal circumstances, and the calcium × phosphate product approximates 30. Intracellularly, cytosolic free phosphate concentrations approximate those in serum—3 to 6 mg/dL.
The serum phosphate concentration is determined by oral intake, intestinal absorption and excretion, renal tubular reabsorption and excretion of filtered phosphate, and release from bone by dissolution of hydroxyapatite by PTH and calcitriol, and fluctuates with age, gender, growth rate, diet, and serum calcium levels (see Fig. 9.2 ). In as much as phosphate is found in all cells and foods, dietary deficiency is unusual. Dietary phosphate is absorbed across the intestinal brush border as HPO 4 −2 in direct proportion to its intake, principally in the duodenum and jejunum. Phosphate is absorbed through the apical membranes of cells lining the small intestine and proximal renal tubule by both passive and active transport pathways. Paracellular transport is a passive mode of phosphate movement from lumen to the extracellular space through the tight junctions between the sides of the epithelial cells that line the small intestine and proximal renal tubule. Phosphate is actively transported across the apical membranes of epithelial cells lining the small intestine through the sodium/phosphate channel designated NPT2b encoded by SLC34A2 (one of three type II sodium/phosphate cotransporters), the sodium-hydrogen exchanger 3 (NHE3 encoded by SLC9A3 ), and the sodium-dependent phosphate transporter encoded by SLC20A2 —members of the type III sodium/phosphate cotransporter family. Expression of SLC34A2 is increased by low dietary phosphate intake and calcitriol and suppressed by PTH. However, PTH also increases intestinal absorption of ingested phosphate (and calcium) by increasing expression of CYP27B1 encoding renal 25-hydroxyvitamin D-1α-hydroxylase, thereby enhancing synthesis of calcitriol, whereas FGF23 has the opposite effect. When dietary phosphate intake falls below 310 mg/d in the adult, net phosphate absorption is negative. At low phosphate intakes, absorption is active in the duodenum, jejunum, and distal ileum, whereas at high intakes, 60% to 80% of ingested phosphate is absorbed primarily by diffusion. Phosphate absorption can be impaired by its intraluminal precipitation as an aluminum or calcium salt and by intestinal malabsorption disorders.
The kidney regulates moment-to-moment phosphate homeostasis. After passage through the renal glomerular membrane, 85% of filtered phosphate is reabsorbed by active transport against an electrochemical gradient across the apical membrane of the cells lining the proximal renal tubule through the type II sodium/phosphate cotransporters encoded by SLC34A1 (NPT2a) and SLC34A3 (NPT2c) with the aid of a Na + , K + -ATPase pump. Phosphate is also reabsorbed to a limited extent in the loop of Henle (10%), distal convoluted tubule (3%), and connecting tubule (2%). Expression of SLC34A1 is increased by hypophosphatemia and suppressed by hyperphosphatemia. PTH, PTHrP, FGF23, and glucocorticoids decrease proximal renal tubular reabsorption of filtered phosphate by lowering expression of SLC34A1 (NPT2a) and SLC34A3 (NPT2c). In the mouse, NPT2a actively transports approximately 70% and NPT2c 30% of proximal renal tubular reabsorbed phosphate; in humans, however, NPT2c may be the major renal tubular transporter of phosphate. The Na + -H + exchanger regulatory factor 1 (NHERF1—encoded by SLC9A3R1 ) is a scaffolding protein that is essential for trafficking of NPT2a and NPT2c to the luminal membrane of cells in the proximal renal tubule; NHERF1 also interacts with PTH1R. The family of phosphate transporters encoded by SLC20A2 (designated PiT2) has also been identified in the renal proximal tubule. Phosphate exits the proximal renal tubular cell with sodium by cation exchange for potassium through channels (encoded by XPR1 ) located on the cell’s basolateral membrane. The maximal tubular reabsorption of phosphate approximates the filtered load. Tubular phosphate reabsorption is increased by low phosphate intake and hypophosphatemia (because of decrease in filtered load), hypercalcemia (by decrease in the glomerular filtration rate), depletion of ECF volume, and metabolic alkalosis. Renal tubular reabsorption of phosphate is depressed by high phosphate intake, PTH (and PTHrP), and the phosphatonin FGF23—and is caused by rapid internalization and subsequent destruction of the NPT2a and NPT2c phosphate cotransporter proteins. The PTH-dependent degradative processes are mediated through the type 1 PTH/PTHrP receptor and transduced by intracellular signaling systems utilizing protein kinase A, protein kinase C, and MAPK. Phosphate is deposited in bone as hydroxyapatite dependent on local levels of calcium, phosphate and alkaline phosphatase activity and reabsorbed by osteoclasts whose activity is stimulated by PTH, calcitriol, and other osteoclast activating factors. There are age-related variations in serum concentrations (mg/dL) of phosphate: less than 5 days, 4.8–8.2; 1–3 years, 3.8–6.5; 4–11 years, 3.7–5.6; 12–15 years, 2.9–5.4; 16–19 years, 2.7–4.7; adult, 2.5–4.5.
Phosphatonins
The phosphatonins are a group of proteins that inhibit renal tubular reabsorption of phosphate. Increasing serum concentrations of phosphate and calcitriol stimulate osteocyte/osteoblast production of the predominant phosphatonin—FGF23—that then depresses synthesis of the phosphate cotransporters NPT2a and NPT2c and decreases renal expression of CYP27BI (encoding 25-hydroxyvitamin D-1α-hydroxylase) and thus formation of calcitriol and consequently, intestinal absorption of calcium and phosphate. Simultaneously, FGF23 enhances expression of renal CYP24A1 (encoding 25-hydroxyvitamin D 24-hydroxylase) and hence the synthesis and urinary excretion of water-soluble 24,25-dihydroxyvitamin D and 1,24,25-trihydroxyvitamin D. FGF23 is generated as a 251 AA protein with a 24 AA signal sequence; for optimal biological function FGF23 must be posttranslationally glycosylated by UDP-N-acetyl-alpha-D-galactosamine:Polypeptide N-acetylgalactosylaminyltransferase 3 (encoded by GALNT3 ). GALNT3 is an enzyme that O-glycosylates the threonine-178 and serine-180 residues of FGF23; failure to glycosylate the threonine-178 site leads to accelerated degradation (and hence inactivation) of FGF23. FGF23 is proteolytically degraded by intracellular subtilisin-like proprotein convertase acting between AA 179 and 180 (… Arg 176 -His 177 -Thr 178 -Arg 179 -Ser 180 …) that separates the amino-terminal FGF-like domain from the carboxyl-terminal tail. Spontaneously occurring gain-of-function mutations within this sequence of FGF23 slow the inactivation of FGF23, thus prolonging its biological life, resulting in autosomal dominant hypophosphatemic rickets (ADHD). FGF23 is expressed primarily by osteoprogenitor cells, osteoblasts, and osteocytes and to a lesser extent by the thymus, brain, thyroid, parathyroid glands, cardiac muscle, liver, and small intestines. In osteoblasts and osteocytes, expression of FGF23 is stimulated by high serum levels or dietary intake of phosphate, PTH, and calcitriol acting through VDR. Inasmuch as calcitriol promotes synthesis of FGF23 whereas FGF23 inhibits production of calcitriol, there is a reciprocal feedback relationship between calcitriol and FGF23.
FGF23 links to the “c” isoform of tyrosine kinase FGF receptors (FGFR) 1, 2, and 3. The genes encoding the FGFRs consist of 19 exons that may be alternatively spliced to include or to exclude either exon 8 or 9 (encoding the third extracellular immunoglobulin-like domain of the FGFR which partially specifies the ligand bound by the receptor); when exon 8 is included in the mRNA transcript the “b” isoform of the FGFR is formed; when exon 9 is included in the transcript, the “c” isoform is produced. FGF23 acts through the dimeric coreceptor quaternary complex—FGF23/αKlotho/FGFR1(IIIc)/heparan sulfate—expressed on the plasma membrane of the target cell to depress renal tubular reabsorption of phosphate, intestinal absorption of phosphate, synthesis of calcitriol, and secretion of PTH. Although it is likely that FGF23 binds to multiple FGFR “c” isoforms, it is the multifunctional transmembrane protein αKlotho (encoded by KL ) that serves as a coreceptor, thereby converting FGFR into specific FGF23 receptors in the parathyroid glands and kidneys.
αKlotho is a 1012 AA protein (encoded by KL whose expression is increased by calcitriol) with extracellular, transmembrane, and intracellular domains synthesized by renal tubular cells that serves as a coreceptor with FGFR1(cIII) for FGF23. Klotho was so named because its loss in mice is associated with a phenotype of premature aging. There are membrane bound and soluble secreted forms of αKlotho; soluble αKlotho is a 549 AA protein composed of the ectodomain of the intact protein and is present in the circulation and excreted by the kidney. The transmembrane form of αKlotho binds FGF23, FGFR1(IIIc), and heparan sulfate to form a quaternary complex that transmits the FGF23 signals that decrease renal tubular cell membrane expression of the genes encoding the phosphate transporters NPT2a ( SLC34A1 ) and NPT2c ( SLC34A3 ), thereby limiting renal tubular reabsorption of filtered phosphate and increasing its urinary excretion. The complex of FGF23/FGFR3,4/αKlotho/heparan sulfate depresses transcription of renal CYP27B1 encoding 25-hydroxyvitamin D-1α hydroxylase necessary for synthesis of calcitriol while enhancing expression of CYP24A1 encoding vitamin D-24 hydroxylase, thereby increasing the solubility and renal excretion of calcitriol and lowering its serum levels.
KL is also expressed in the parathyroid gland; there, FGF23 interacting with αKlotho and FGFR3,4 decreases transcription of PTH and thereby reduces PTH synthesis and secretion. Reciprocally, both calcitriol and PTH increase synthesis of FGF23 by bone cells, establishing a feedback loop regulating FGF23 synthesis and secretion. After binding of the FGF ligand to the FGFR, the receptors dimerize, autophosphorylate, and then phosphorylate intracellular proteins involved in signal transduction, including PLCγ, growth factor receptor bound (GRB)-14, son of sevenless, FGF receptor substrate (FRS)-2—a docking protein that links the activated FGFR to the phosphoinositide-3-kinase (PI3K), and MAPK signaling pathways. FGF23 expression is increased by iron deficiency by interrupting the negative feedback effect of hypophosphatemia upon FGF23 synthesis. The truncated, alternatively spliced form of αKlotho with 549 AA is secreted into the circulation, cerebrospinal fluid, and urine. Soluble αKlotho regulates signal transduction by growth factors (insulin, insulin-like growth factor-1) independently of FGF23 through binding to sialogangliosides—components of intramembranous lipid rafts. Soluble αKlotho enhances renal calcium absorption by cells lining the renal distal convoluted and connecting tubules by increasing the number of calcium transport channels encoded by TRPV5 expressed on the apical membrane of these cells.
Serum values of FGF23 are increased in patients with X-linked hypophosphatemic rickets, autosomal recessive and dominant hypophosphatemic rickets, tumor-induced osteomalacia, and fibrous dysplasia associated with hypophosphatemia. In patients with autosomal dominant hypophosphatemic rickets, gain-of-function mutations (e.g., Arg179Trp) in FGF23 result in resistance to degradation of FGF23, a protein that is normally cleaved between Arg179 and Ser180, whereas in subjects with tumor induced osteomalacia, the rate of production of FGF23 is greatly increased. In familial tumoral calcinosis (OMIM 211900), loss-of-function mutations in FGF23 lead to accelerated intracellular degradation of FGF23 that prevents secretion of intact protein resulting in decreased renal excretion of phosphate, hyperphosphatemia, relatively increased calcitriol levels, and diffuse ectopic calcification.
Phosphatonins were first identified by analysis of tumors associated with hypophosphatemic osteomalacia. Among other phosphatonins are matrix extracellular phosphoglycoprotein (encoded by MEPE ) and secreted frizzled-related protein 4 (encoded by SFRP4 ). MEPE is expressed by osteoblasts, osteocytes, and odontoblasts. Expression of MEPE is inhibited by calcitriol. MEPE is a 525 AA, 58-kDa protein that is a member of the short-integrin-binding, ligand-interacting glycoprotein family whose genes are clustered on chromosome 4q22.1. MEPE modulates osteoblast and osteoclast function and may both inhibit and support bone mineralization. MEPE enhances phosphaturia by increasing expression of FGF23 . MEPE (and other phosphatonins) contains within its structure an acidic serine aspartate-rich MEPE-associated (ASARM) peptide that may be released from its parent structure by cathepsins B and K in phosphorylated or nonphosphorylated forms. Phosphorylated ASARM and MEPE bind avidly to hydroxyapatite and prevent further deposition of calcium and phosphate; ASARM also inhibits phosphate uptake by the intestinal tract and the renal tubule (MEPE also is involved in the osteocytic response to a mechanical load). MEPE and ASARM are substrates for the X-linked phosphate-regulating endopeptidase (encoded by PHEX ); inactivating variants of PHEX result in X-linked hypophosphatemic rickets.
Other SIBLING noncollagenous matrix proteins include dentin-matrix acidic phosphoprotein 1 (encoded by DMP1 ), dentin sialo-phosphoprotein ( DSPP ), osteopontin (also termed secreted phosphoprotein 1 [SPP1]) , and integrin-binding sialoprotein ( IBSP ). In addition to sharing the ASARM sequence near their carboxyl terminals, these proteins have in common an Arg-Gly-Asp (RGD) tripeptide motif that interacts with and binds to integrins on the surface of interacting cells. The basic ASARM motif consists of 23 AA; several of its serine residues are specifically phosphorylated by casein serine kinase. Phosphorylated and nonphosphorylated ASARMs released by DMP1, DSPP, and SPP1 are also resistant to proteolysis, except by the zinc metalloendopeptidase PHEX. Inactivating mutations of PHEX results in X-linked hypophosphatemic rickets, in part, by inability to degrade the ASARM peptide coating of hydroxyapatite. PHEX may also be involved in the expression of FGF23 and the stability of FGF23. Carboxyl to the ASARM motif of DMP1 is an additional sequence of 35 AA that is termed the minfostin motif; a mutation in this region of DMP1 results in one form of autosomal recessive hypophosphatemic rickets. The ASARM region of DMP1 binds to PHEX sited on the plasma membrane of the normal osteocyte suppressing expression of PHEX ; displacement of binding by excessive free ASARM peptides (as in patients with hypophosphatemic rickets) further increases FGF23 expression. In addition to patients with tumor-induced osteomalacia and X-linked hypophosphatemic rickets, increased serum concentrations of FGF23 are found in subjects with autosomal dominant hypophosphatemic rickets because of activating mutations in FGF23 and autosomal recessive hypophosphatemic rickets types 1 and 2 because of loss-of-function mutations in DMP1 or ENPP1 , respectively.
Secreted Frizzled-related protein 4 (FRP4) is a 346 AA glycosylated protein that shares the structure of the extracellular domain of the transmembrane Frizzled GPCR. The natural ligands of Frizzled receptors are wingless (WNT) proteins, and their coreceptors are cell-surface low-density lipoprotein receptor-related proteins; these heterotrimeric complexes stabilize intracellular β-catenin and the attendant signal transduction systems that are essential for many transformative processes including cartilage and bone formation. Secreted FRP4 serves as a “decoy” receptor for WNT proteins and thereby competes with the Fizzled receptor for WNT binding and by so doing inhibits the function of the Frizzled receptor. FRP4 is expressed in bone cells and in large amounts by tumors with associated osteomalacia. FRP4 inhibits sodium-dependent renal tubular phosphate reabsorption by inhibition of WNT signaling leading to hypophosphatemia; it also reduces expression of CYP27B1 , the gene encoding 25-hydroxyvitamin D 3 -1α.hydroxylase.
Immunoreactive species of FGF23 are measurable in normal adult sera but values vary depending on the epitopes recognized by the antibodies used, that is, whether they are specific for the full-length FGF23 protein or only for its carboxyl-terminal fragment, and on the assay methodology (e.g., enzyme-linked immunosorbent assay or chemiluminescent immunoassay). In assays that recognize full-length FGF23 and carboxyl-terminal fragments of FGF23, intact FGF23 values vary diurnally and there is marked interindividual variability in FGF23 levels. The serum FGF23 concentration is inversely related to that of phosphate, and values rise when dietary phosphate increases (or during infusion of phosphate) and decline with phosphate restriction.
Magnesium
Magnesium (Mg 2 + ) is the fourth most abundant of the body cations. It is an intracellular and extracellular divalent cation that is critical for DNA and protein synthesis, neuroexcitability, intracellular signaling, oxidative phosphorylation, and bone hydroxyapatite development. In serum, magnesium is present in the bound and free states. Approximately 1% of body magnesium is present in the ECF compartment, 14% in muscle and soft tissue, and 85% in bone, where it is found on the surface of the hydroxyapatite crystal and of which 50% is freely exchangeable. In blood, magnesium (1.7–2.4 mg/dL = 0.7–1.0 mmol/L) is partially bound to proteins (approximately 30%), complexed to phosphate and other anions (15%), and free Mg 2 + (55%). As with calcium, magnesium levels rise as pH declines (with increased acidity). Intracellularly, magnesium (0.5 mmol/L) is bound to ATP and other molecules; 10% is in the ionic form and 60% is within mitochondria. Mg 2 + is a cofactor in many enzymatic reactions, including those that consume or produce ATP. Magnesium alters free radicals and influences nitric oxide synthase, cyclic guanosine monophosphate generation, endothelin production, and immune function. Magnesium decreases membrane excitability in nerve and muscle cells and blocks the excitatory N-methyl D-aspartate receptor. It is a necessary cofactor for the regulation of neuromuscular excitability, nerve conduction, oxidative metabolism by mitochondria, glycolysis, phosphorylation, and transcription and translation of DNA. It is essential for the secretion (but not the synthesis) of PTH by the parathyroid chief cell.
Net intestinal magnesium absorption is directly related to dietary intake and independent of calcitriol. The bulk of ingested magnesium is absorbed in the small intestine (and colon) in proportion to the intestinal luminal concentration of this cation through voltage-dependent paracellular channels within tight intercellular junctions, a process assisted by claudins. There is a small component of active, transcellular intestinal magnesium absorption through ion channels encoded by transient receptor potential cation channel, subfamily M, member 6 ( TRPM6 ) linked to the transcript of TRPM7 , a magnesium-permeable cation channel. The TRPM6/TRPM7 heterooligomer is expressed on the surface of cells lining the intestinal tract. Loss-of-function mutations in TRPM6 result in autosomal recessive hypomagnesemia with secondary hypocalcemia because of impairment of intestinal (and renal tubular) absorption of this cation. The intestinal absorption of magnesium may be impaired by high phosphate intake, intestinal dysfunction, or chronic laxative abuse. Magnesium is also excreted into the intestinal tract.
Some 70% of serum magnesium is ultrafiltrable and passes through the renal glomerular membrane; approximately 95% of filtered magnesium is reabsorbed—15% in the proximal renal tubule, 70% in the cortical TALH, and 10% in the distal convoluted tubule. Thus 3% to 5% of filtered magnesium is excreted in the urine. In the proximal renal tubule and TALH, magnesium reabsorption is primarily passive through paracellular tight junction channels using claudins 10, 16, and 19. In the proximal renal tubule, reabsorption of water increases intratubular magnesium concentration, thereby facilitating its paracellular reabsorption. Tight junction claudin proteins bridge intercellular gaps and regulate the movement of ions across an epithelial sheet. Claudin 10 (encoded by CLDN10 ) is a 226/228 AA protein. Biallelic inactivating mutations in CLDN10 result in the HELIX syndrome (OMIM 617671) of anhidrosis, alacrima, xerostomia, malformed teeth, hypermagnesemia, renal wasting of sodium and chloride, and secondary hyperparathyroidism. Paracellin-1 (encoded by CLDN16 ) is a 305 AA protein expressed in the renal cortical TALH and distal convoluted tubule; paracellin-1 has four transmembrane domains with intracellular carboxyl and amino terminals. Paracellin-1 is also employed for paracellular reabsorption of calcium in the TALH. Homozygous or compound heterozygous loss-of-function mutations of CLDN16 lead to familial, autosomal recessive renal hypomagnesemia (type 3) characterized by renal wastage of magnesium in association with hypercalciuria and nephrocalcinosis (OMIM 248250). Claudin 19 is a 224 AA tight junction protein. Biallelic inactivating mutations in CLDN19 result in renal wasting of magnesium, hypomagnesemia, hypercalciuria, and nephrocalcinosis, leading to renal failure (hypomagnesemia type 5—OMIM 248190); in addition, because CLDN19 is expressed in the eye, affected subjects have decreased vision because of retinal malformation.
In the TALH, magnesium reabsorption occurs through a paracellular pathway that is impermeable to water and is propelled by a transepithelial voltage gradient that is lumen-positive and generated by a Na + -K + -ATPase. The voltage gradient requires the integrated action of a Na + -K + -Cl − -coupled cotransporter (NKCC2 encoded by SLC12A1 ), a Cl − channel (CLC-Kb encoded by CLCNKB ), and a K + channel (ROMK encoded by KCNJ1 ). Barttin, encoded by BSND , is an essential beta subunit of the CLCNKB Cl − channel. Active transcellular movement of magnesium through the TALH is accomplished utilizing the NKCC2 cotransporter, a pathway inhibited by activation of the CaSR. In the renal distal convoluted tubule, magnesium is actively reabsorbed through a channel composed of a heterooligomer of TRPM6 linked to TRPM7 expressed on the surface of cells lining this renal segment. It is primarily in the distal convoluted tubule that magnesium is actively transported through apical (luminal) membrane TRPM6 channels. The renal tubular expression of TRPM6 and intracellular movement of TRPM6 is regulated by dietary magnesium, estrogens, acid-base balance, and proepidermal growth factor (pro-EGF). TRPM7 is also expressed on the luminal surface of renal cells in the distal convoluted tubule where it too facilitates reabsorption of magnesium. Other magnesiotropic proteins expressed in the distal convoluted tubule are the thiazide sensitive Na + :Cl − cotransporter, potassium channels Kv1.1 and Kir4.1, and hepatocyte nuclear factor 1B (HNF1B). Biallelic inactivating mutations in TRPM6 result in hypomagnesemia type 1 with secondary hypocalcemia (OMIM 602014); this disorder presents in early infancy with tetany and seizures and is caused by both impaired absorption of intestinal magnesium and reabsorption of renal tubular magnesium. Pro-EGF regulates the availability and activity of TRPM6 by enabling its movement from intracellular sites of storage to the luminal plasma membrane of renal distal convoluted tubular cells and colonic epithelial cells; biallelic loss of EGF leads to hypomagnesemia type 4 (OMIM 611718). Loss of SLC12A3 and its encoded product—the thiazide sensitive Na + :Cl − cotransporter—leads to reduced TRPM6 activity or abundance and the Gitelman syndrome (OMIM 263800) of hypomagnesemia, hypocalciuria, and hypokalemic metabolic alkalosis. Loss of the voltage-gated K + channel Kv1.1 (encoded by KCNA1 ) that colocalizes with TRPM6 alters the polarization of the renal epithelial cell of the distal convoluted tubule, a functional abnormality that impairs activity of TRPM6 and hence of magnesium transport resulting in autosomal dominant hypomagnesemia with episodic ataxia, tetany, muscular cramping, and myokymia (involuntary, localized quivering of a few muscle fibers within a muscle) (OMIM 160120). The pathogenesis of hypomagnesemia (OMIM 612780) that results from the loss-of-function mutations in KCNJ10 encoding the inwardly rectifying K + channel—Kir4.1—is similar to that described for loss of KCNA1 . Inactivating mutations of KCNJ10 lead not only to hypomagnesemia but are also associated with the SESAME syndrome (seizures, sensory neural deafness, ataxia, mental retardation, electrolyte imbalance; OMIM 612780).
PTH increases magnesium reabsorption in the renal TALH and distal convoluted tubule; hypermagnesemia and hypercalcemia (acting through the CaSR) decrease renal tubular magnesium reabsorption as do expansion of ECF volume, metabolic alkalosis, phosphate depletion, loop diuretics, aminoglycoside antibiotics, and impaired renal function.
Alkaline phosphatase
The alkaline phosphatases are a group of transmembrane glycoproteins tethered to cell plasma membranes that remove phosphate groups from organic substrates; the extracellular (ecto) domain may be released from the cell membrane into the circulation by a phospholipase. There are three tissue-specific alkaline phosphatases (placental, germ cell, intestinal) and a tissue nonspecific alkaline phosphatase (TNSALP) (encoded by ALPL ), the latter composed of several isoforms with similar protein composition of 507to 524 AA but differing carbohydrate configurations that are present in the skeleton, teeth, liver, and kidney. Bone TNSALP is generated by chondroblasts and osteoblasts by alternative processing of ALPL utilizing one of two leading exons during transcription and translation, permitting the osteoblast to synthesize and release a specific bone isoform. Functional as a homodimer, TNSALP removes phosphate residues (organically bound to a serine residue) from many phosphoprotein substrates including inorganic pyrophosphate, a substance that inhibits mineralization of bone organic matrix, phosphoethanolamine, and pyridoxyl 5′-phosphate, the precursor of pyridoxine in the absence of which seizures recalcitrant to treatment may develop.
Although TNSALP circulates as a homodimer, in tissue, it is a homotetrameric ectoenzyme (ectophosphatase) located on the cell surface of the osteoblast anchored through its carboxyl terminal to cell membrane phosphatidylinositol-glycan. In bone, alkaline phosphatase: (1) binds to collagen type I and prepares skeletal matrix for mineralization; (2) transports inorganic phosphate and free calcium into the cell; and (3) hydrolyzes organic phosphates, principally pyrophosphate and phosphoethanolamine, thereby increasing the local concentration of phosphate to values that exceed the calcium × phosphate product encouraging deposition of calcium phosphate as hydroxyapatite on collagen type I fibers. Inactivation of pyrophosphate and other inhibitors of mineralization (e.g., phosphorylated ASARM derived from SIBLING proteins by removing their phosphate moieties by PHEX) permits formation of hydroxyapatite. The hepatic form of TNSALP is formed by alternative splicing of dual exons 1 of ALPL . Hepatic alkaline phosphatase converts pyridoxyl-5′-phosphate (the major circulating form of vitamin B 6 ) to pyridoxal, a compound essential for normal synthesis of neural GABA, an inhibitory neurotransmitter; without pyridoxal-5′-phosphate central nervous system levels of GABA are low and seizures may occur. Loss-of-function mutations in ALPL lead to infantile, childhood, and adult forms of hypophosphatasia. The three genes encoding tissue-specific intestinal, placental, and germ cell alkaline phosphatase isoenzymes are clustered on chromosome 2q34-q37.
Parathyroid hormone; parathyroid hormone-related protein; PTH/PTHRP receptors
Parathyroid Hormone
PTH increases concentrations of plasma Ca 2 + , lowers serum phosphate values, stimulates synthesis of calcitriol, and exerts both anabolic and catabolic effects on bone. The chief cells of four paired parathyroid glands are derived from the endoderm of the dorsal segments of the third (paired inferior glands) and fourth (paired superior glands) pharyngeal pouches; occasionally, there may be a fifth parathyroid gland embedded within the substance of the thyroid gland or in the mediastinum. The thymus is formed by the endoderm of the third pharyngeal pouch and calcitonin-synthesizing parafollicular C cells of the thyroid by endoderm of the fourth pharyngeal pouch. Transcription factors required for the development of the pharyngeal pouches and differentiation and organization of the parathyroid glands include T-box 1 (encoded by TBX1 ), GATA-binding factor/protein 3 (encoded by GATA3 ), glial cell missing, drosophila, homolog 2 (encoded by GCM2 ), and V-MAF musculoaponeurotic fibrosarcoma oncogene family, protein B (encoded by MAFB ), a factor that aids in the movement of the parathyroid glands to sites on the periphery of the thyroid gland ( Fig. 9.3 ). GATA3, GCM2, and MAFB are essential for the expression of PTH and synthesis of PTH postnatally. Variants of GATA3 are associated with the syndrome of hypoparathyroidism, sensorineural deafness, and renal disease (OMIM 146255), whereas mutations in GCM2 result in either familial hypo- or hyperparathyroidism (OMIM 146200/617413, respectively). In addition, four-and-a-half Lim domains (encoded by FHL1 ) is a gene that is also required for parathyroid gland formation as an inactivating variant of this gene is associated with aplasia of these structures. PTH is composed of three exons; exon 1 is transcribed but not translated; the second and third exons of PTH encode the prepro-PTH sequence of 115 AA that is processed to intact 84 AA PTH that is released from the parathyroid glands in response to declining serum concentrations of Ca 2 + , detected by the CaSR expressed on the plasma membrane of the parathyroid gland chief cell.
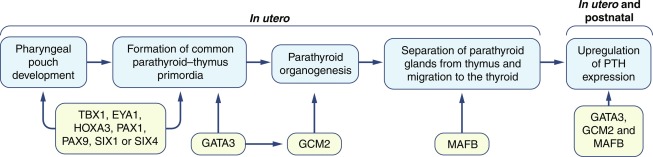
PTH is recognized by the heptahelical PTH1R GPCR expressed on the plasma membranes of target tissue cells, including those that line the intestinal tract and renal tubules. In the distal renal tubule and collecting ducts, PTH increases reabsorption of filtered calcium. PTH inhibits reabsorption of filtered phosphate in the proximal renal tubule. PTH also stimulates expression of CYP27B1 encoding renal 25-hydroxyvitamin D-1-alpha hydroxylase and consequently renal synthesis of calcitriol. In the intestinal tract, calcitriol increases absorption of calcium. PTH stimulates osteoblastogenesis and indirectly osteoclastogenesis, the latter by enhancing osteoblast expression of TNFSF11 encoding the receptor activator of NF-κB–ligand (RANK-ligand) and suppressing that of TNFRSF11B encoding osteoprotegerin, a decoy receptor for RANK-ligand. The intracellular message of PTH is transmitted by activation of signal transduction systems, including the cyclic AMP–protein kinase A, calcium-related protein kinase C, and MAPK pathways and those utilizing phospholipases A and C.
The synthesis and secretion of PTH by parathyroid gland chief cells is regulated by Ca 2 + concentrations acting through the CaSR that either enhance (when Ca 2 + concentrations are low) or repress (when they are high) transcription of PTH and secretion of PTH; Ca 2 + values also modulate the rate of chief cell proliferation, a response also mediated by the CaSR. Calcimimetic drugs have inhibitory effects on PTH secretion, a property that has made these agents useful in the management of patients with excess secretion of PTH, such as mild hyperparathyroidism and autosomal dominant familial hypocalciuric hypercalcemia. Low serum concentrations of phosphate inhibit transcription of PTH , whereas rising serum phosphate values enhance production of PTH not only indirectly by lowering of Ca 2 + values but also by stabilizing PTH mRNA, permitting greater translation of PTH and by stimulation of chief cell replication. Calcitriol inhibits transcription of PTH , secretion of PTH, and proliferation of chief cells; FGF23 also decreases PTH secretion. In response to acute hypocalcemia PTH stored in secretory vesicles is rapidly released. When hypocalcemia is prolonged, the secretion of PTH 1-84 is augmented by decrease in its rate of intracellular degradation and increase in the rate of transcription of PTH ; when hypocalcemia is extended PTH synthesis and secretion are amplified by increase in chief cell number. When Ca 2 + values rise, the secretion of PTH is decreased acutely by degradation of stored PTH 1-84 by calcium-responsive proteases within the parathyroid glands; if prolonged, hypercalcemia leads to decrease in expression of PTH and to decline in the number of chief cells.
The amino-terminal 25 AA signal sequence (encoded by exon 2) of 115 AA prepro-PTH is removed by furin, a prohormone convertase, as the polypeptide leaves the endoplasmic reticulum forming the 90 AA pro-PTH (encoded by exon 3) that is then further processed in the Golgi apparatus by furin to mature human 84 AA PTH 1-84 that is stored in secretory vesicles and granules and from which it is either secreted by an exocytic mechanism or degraded by calcium-sensitive proteases (cathepsins B and H colocalized within the vesicles) to smaller amino and carboxyl-terminal fragments of PTH. PTH degradation represents an important mechanism that regulates the release of intact bioactive PTH 1-84 and is accelerated when the plasma Ca 2 + concentration is elevated. The full biological activity of PTH 1-84 is found in its first 34 AA sequence and all of its skeletal and renal affects (vide infra) are localized within the segment PTH 1-31 . Amino acids numbers 1 and 2 (ser-val) comprise an activation sequence that is essential for bioactivity of the amino-terminal portion of PTH 1-84 . Amino acids 20 to 26 constitute a core sequence necessary for binding to the amino-terminal ectodomain of PTHR1; within this seven AA sequence, Arg and Trp are critically important for binding of the PTH ligand to its receptor. In addition to intact PTH 1-84 , the parathyroid glands secrete a phosphorylated form of PTH 1-84 and carboxyl-terminal PTH fragments of varying length, but they do not secrete amino-terminal fragments of PTH 1-84 . The half-life of circulating PTH 1-84 is approximately 2 minutes; it is rapidly metabolized by the liver and excreted by the kidney. In the hepatic Kupffer cells, PTH 1-84 is cleaved usually either between AA 33 and 34 or between AA 36 and 37 to carboxyl-terminal PTH peptides with half-lives of approximately 15 to 20 minutes. In the kidney, intact and carboxyl-terminal fragments of PTH are filtered by the glomerulus, reabsorbed across the apical membrane of proximal renal tubular cells, and then degraded to smaller fragments. Megalin, a multifunctional low-density lipoprotein-related receptor (encoded by LRP2 ) expressed in coated pits on the luminal/apical surfaces (as well as endocytic vacuoles and lysosomes) of proximal renal tubular cells, specifically recognizes intact PTH 1-84 and fragments of PTH and mediates the renal tubular endocytosis of PTH 1-84 that has been filtered through the glomerulus.
The classical functions of PTH 1-84 , as well as its shorter amino peptide derivatives PTH 1-34 and PTH 1-31 upon regulation of calcium and phosphate homeostasis are carried out through the seven transmembrane, G-protein–coupled type 1 PTH/PTHrP receptor sited on cell membranes in the renal tubule and osteoblast. Thus with equal potency PTH 1-84 , PTH 1-34 , and PTH 1-31 : (1) inhibit renal tubular reabsorption of phosphate, thereby increasing its urinary excretion; (2) increase renal tubular reabsorption of Ca 2 + ; (3) augment renal synthesis of calcitriol by enhancing expression of CYP27B1 (encoding 25-hydroxyvitamin D 3 -1α hydroxylase), thereby amplifying intestinal absorption of calcium; (4) stimulate osteoblast production of RANK-ligand, thus enhancing osteoclastogenesis and osteoclastic reabsorption of calcium; and (5) mediate the anabolic effects of PTH on the volume and microarchitecture of bone. PTH 1-84 , PTH 1-34 , and PTH 1-31 stimulate intracellular signal transduction through several G-protein–coupled pathways, including the G sα -adenylyl cyclase-cyclic AMP-PKA and the G q/11 -PLC-β-inositol triphosphate (IP 3 )/DAG-Ca 2 + -protein kinase C (PKC)-MAPK signal transduction pathways. PTH 1-84 and PTH 1-34 also induce cell function through β-arrestin that does not involve a G-protein that in turn activates intracellular signaling pathways mediated by MAPK, protein kinase B (PKB), and PI3K.
Multiple carboxyl-terminal peptides derived from PTH 1-84 are either secreted directly by the parathyroid chief cells or return to the circulation after metabolism of intact PTH 1-84 by hepatic Kupffer cells. Indeed, carboxyl-terminal fragments of PTH 1-84 are secreted in greater abundance from the parathyroid glands than is intact PTH 1-84 , and the proportion of carboxyl-terminal fragments secreted increases as the ambient Ca 2 + concentration rises. Amino-terminal fragments generated by hepatic catabolism of PTH 1-84 are degraded further within the liver and do not recirculate. PTH 1-84 and the carboxyl-terminal fragments of PTH are filtered by the kidneys; the carboxyl-terminal fragments are reabsorbed by the renal tubules and further degraded intracellularly. Among the circulating carboxyl-terminal fragments of PTH are PTH 7-84, 24-84, 34-84, 37-84,41-84, 43-84 .
In addition to their classical effects on mineral homeostasis, several nonclassical actions of PTH 1-84 have been identified including: (1) rapid and direct stimulation of intestinal calcium absorption independent of its effects on vitamin D metabolism, (2) stimulation of hepatic gluconeogenesis, (3) acute natriuresis and calciuresis, and (4) enhancement of neutrophil movement in vitro. Inasmuch as many of these nonclassical biological effects of intact PTH are not replicated by the amino–terminal fragment PTH 1-34 , it has been suggested that they may be related to the carboxyl-terminal portion of the protein. Indeed, the carboxyl-terminal fragments of PTH may have biological functions that oppose those of the amino-terminal portion of PTH, namely, hypocalcemic properties that are perhaps mediated by proapoptotic effects on both osteoclasts and osteocytes.
Because there are multiple circulating forms of PTH, its immunological measurement is dependent on the specificity of the antibody(ies) used in the assay. When a “first-generation” polyclonal PTH radioimmunoassay is used, both intact and carboxyl-terminal fragments of PTH are usually measured. Use of “second-generation” immunoradiometric and immunochemoluminometric assays using dual monoclonal antibodies, the first directed toward an epitope within the amino terminal of PTH (AA 20–25) and the second specific for an epitope in the carboxyl terminal of PTH (e.g., AA 59–78), improves the specificity of immunological assays for intact PTH 1-84 . Nevertheless, most of these assays detect both intact and selected carboxyl-terminal fragments of PTH and consequently measured PTH values may be inappropriately high, particularly in patients with chronic renal insufficiency in whom circulating levels of carboxyl-terminal fragments of PTH are high. Furthermore, the comparability of PTH assays from different commercial sources is often limited. Using a two-site immunochemiluminescent assay, serum PTH concentrations average approximately 11 to 13 pg/mL and range between 2.3 and 24.5 pg/mL in children and adolescents 2 to 16 years of age; values do not vary with age but are a bit higher in girls than boys. Development of “third-generation” PTH assays that use antibodies that are directed to an epitope of the first four amino acids of PTH has improved the specificity and stability of assays for PTH 1-84 . A “fourth generation” of PTH assays uses immunoaffinity, in situ digestion, liquid chromatography, and tandem mass spectrometry to separate PTH 1-84 from smaller PTH fragments.
The synthesis and secretion of PTH 1-84 and its various fragments are modulated for the most part by the serum Ca 2 + concentration acting through the CaSR expressed on the plasma membrane of the parathyroid chief cell. Rapidly declining, as well as steady-state low serum concentrations of Ca 2 + , increase PTH secretion by accelerating its release from storage sites in secretory vesicles; hypocalcemia also raises intracellular levels of PTH mRNA by increasing the transcription rate of PTH and enhancing the stability of PTH mRNA by its posttranscriptional binding to cytosolic proteins. Hypercalcemia deceases PTH transcription and destabilizes and thence lowers cellular levels of PTH mRNA. The serum Ca 2 + concentration also determines the form of PTH released by the parathyroid gland; during hypocalcemia PTH 1-84 is the predominant form secreted; in hypercalcemic states, carboxyl-terminal fragments of PTH are released in abundance.
Low serum phosphate concentrations exert an independent and direct inhibitory effect on the transcription of PTH , posttranscriptional PTH mRNA stability, PTH secretion, and proliferation of parathyroid chief cells; hyperphosphatemia increases PTH secretion by lowering plasma concentrations of Ca 2 + and by enhancing the stability of PTH mRNA. Prolonged hyperphosphatemia contributes to hyperplasia of the parathyroid glands frequently encountered in patients with chronic renal disease. Calcitriol directly inhibits PTH transcription acting through the nuclear VDR. Calcitriol also controls expression of CASR and of VDR and decreases proliferation of parathyroid cells. However, chronic hypocalcemia overcomes the suppressive effects of calcitriol on PTH transcription by decreasing VDR number in the parathyroid chief cell. FGF23 released by osteocytes and osteoblasts directly impedes synthesis and secretion of PTH. Both hypomagnesemia and hypermagnesemia inhibit release but not synthesis of PTH. Other agents that increase PTH release include: β-adrenergic agonists, dopamine, prostaglandin E, potassium (by decreasing cytosolic Ca 2 + levels within the parathyroid chief cell), prolactin, lithium (by “resetting” the set-point for PTH release), glucocorticoids, estrogens, and progestins. Prostaglandin F 2α , α-adrenergic agonists, and fluoride suppress PTH release by increasing cytosolic Ca 2 + values.
PTH regulates the serum concentration of calcium by stimulating its reabsorption in the distal renal tubule and from the skeleton and indirectly by augmenting the intestinal absorption of calcium by increasing the synthesis of calcitriol. In bone, PTH enhances osteoclast differentiation and maturation indirectly by acting on and through stromal cells and osteoblasts to increase synthesis of RANK-ligand, an activator of osteoclastogenesis, and to suppress that of osteoprotegerin, a decoy receptor for RANK-ligand. When administered intermittently, however, PTH 1-84 and PTH 1-34 exert anabolic effects upon skeletal mass by increasing osteoblast number by accelerating their differentiation from progenitor cells and from inactive bone-lining cells. They do so in part by decreasing the stimulatory effects of the peroxisome proliferator activator receptor gamma (PPARγ) upon mesenchymal stem cell differentiation into adipocytes permitting their differentiation into osteoblasts, by augmenting the rate of osteoblast proliferation, and by reducing the rate of apoptosis of osteoblasts. The anabolic effects of PTH on bone formation are further mediated by enhancing synthesis and release of matrix-embedded growth factors, such as locally generated insulin-like growth factor-I (IGF-I), which has positive effects on differentiation and survival of osteoblasts, by stimulating the WNT-Frizzled-β-Catenin pathway of osteoblastogenesis, and by inhibiting antagonists to bone formation, such as sclerostin. In addition, through promotion of β-arrestin–mediated intracellular signal transduction, PTH increases trabecular number and volume. Thus PTH stimulates anabolism of bone mineralization whereas bisphosphonates exert an antiresorptive effect. PTH stimulates calcitriol synthesis by increasing renal tubular expression of CYP27B1 , the gene encoding 25-hydroxyvitamin D 3 -1α-hydroxylase, the enzyme that catalyzes the synthesis of calcitriol from calcidiol. PTH depresses proximal and distal renal tubular reabsorption of phosphate by decreasing expression of SLC34A1 , encoding a Na + -HPO 4 cotransporter, thus increasing the urinary excretion of this anion. In addition, PTH directly stimulates secretion of bone-derived FGF23, thereby further increasing urinary phosphate excretion; PTH also indirectly stimulates FGF23 release by lowering serum phosphate concentrations and increasing those of calcitriol.
Parathyroid Hormone-Related Protein
PTHrP also termed PTH-like protein ( PTHLP ) (encoded by PTHLH ) was initially identified as a major cause of humoral hypercalcemia of malignancy. PTH and PTHrP have evolved from a common ancestral gene through gene duplication, and they are structurally and functionally related. Thus the sequence of AA in the preproportion of the translated products and the first eight of 13 AA at the beginning of their first coding exons are identical; although their AA sequences diverge thereafter, the predicted three-dimensional structures of the next 21 AA of both peptides are similar, as is the PTH1R to which the two proteins bind and through which they transmit their intracellular signals. However, PTH binds more avidly to PTHR1 and elicits a greater hypercalcemic response than does PTHrP. Following transcription, PTHLH is translated into isoforms composed of the first 139, 141, and 178 AA. PTHrP transmits its message both distally as an endocrine hormone and locally as both a paracrine and intracrine messenger. Variable parts of PTHrP are also secreted by keratinocytes, epithelial cells of the breast, and other tissues in sequences that include: AA 1 to 36 (which binds to and activates PTH1R), AA 38 to 94, and AA 107 to 138. Acting through the vitamin D nuclear receptor, calcitriol inhibits transcription of PTHLH and decreases the stability of PTHrP mRNA, thereby increasing the rate of intracellular degradation of the PTHrP protein.
Because transcription of PTHLH may begin in an exon downstream of the exon that encodes its signal peptide, after synthesis PTHrP can remain in the cytoplasm and be directly imported into the nucleus. A nuclear localization sequence is present between AA 84 and 93 in the PTHrP molecule. After synthesis, PTHrP retained within the cytoplasm of the synthesizing cell is bound to importin β1 and shuttled between cytosol and nucleus where it links to and regulates movement of targeted (m)RNA into the ribosome and translation of the selected gene(s), thus serving as an intracrine signaling transducer. PTHrP also transmits its message in a paracrine manner between cells within tissues. PTHrP is synthesized by many fetal and adult tissues (cartilage, bone, smooth, cardiac and skeletal muscle, skin, breast, intestines, parathyroid glands, pancreatic islets, pituitary, placenta, and central nervous system) and plays a crucial role in chondrocyte differentiation and maturation, formation of the mammary gland and eruption of teeth, and epidermal and hair follicle growth. In the fetus, periarticular chondrocytes located at the ends of long bones synthesize PTHrP in response to Indian hedgehog (encoded by IHH ), a protein secreted by late proliferative-early prehypertrophic chondrocytes that functions through its receptor—Patched 1 (encoded by PTCH1 ). PTHrP enters into and diffuses through the growth plate and signals prehypertrophic chondrocytes through PTH1R to slow their rate of differentiation to hypertrophic chondrocytes—thus prolonging the stage of chondrocyte proliferation and delaying ossification, thereby increasing the length of the cartilaginous growth plate and the long bone. In addition to stimulating the secretion of PTHrP, the rate of growth plate chondrocyte proliferation is increased by IHH, which also directs differentiation of perichondrial cells to osteoblasts. PTHrP additionally influences osteoblast-induced bone formation and tooth eruption. PTHrP secreted by the fetal parathyroid gland and the PTHrP 37-94 midregion fragment synthesized by the placenta increase placental calcium transport ( Table 9.3 ). Both PTH and PTHrP are required for normal mineral homeostasis in the fetus.
Site | Action |
---|---|
Mesenchyma | |
Subarticular cells/prechondrocytes | PTHrP depresses the rate of differentiation of late proliferating chondrocytes to hypertrophic chondrocytes and delays ossification, thus permitting increased longitudinal growth of the cartilaginous growth plate |
Bone | Enhances or depresses bone resorption |
Smooth muscle | Relaxation |
Vascular system | |
Myometrium | |
Urinary bladder | |
Cardiac muscle | Positive chronotropic and inotropic effects |
Skeletal muscle | |
Epithelia | |
Skin | Regulates proliferation of keratinocytes |
Breast | Induces ductal branching and formation of breast epithelium, secreted into milk, drives mobilization of calcium from maternal bone for transfer to nursing infant |
Teeth | Stimulates resorption of overlying bone enabling eruption |
Endocrine system | |
Parathyroid glands | Stimulates transport of calcium |
Pancreatic islets | Stored and cosecreted with insulin |
Placenta | Enhances calcium transport from mother to fetus |
Central nervous system | Neuroprotective by antagonizing excessive calcium |
Normal nipple and mammary gland formation is dependent upon local synthesis of PTHrP. In utero, this protein promotes differentiation of mesenchymal tissue surrounding the budding epithelial mammary ducts; PTHrP is also important for terminal differentiation of the mammary system during puberty. During lactation, mammary expression of PTHLH and secretion of PTHrP increases while production of estrogens declines permitting unopposed PTHrP-induced mobilization of maternal skeletal calcium necessary to meet the calcium requirement of the breastfed infant but substantially decreasing maternal bone mineral content, a process that is reversed when lactation ceases.
Biallelic loss of PTHrP in “knock-out” mice is lethal because of bony malformations. In Pthlh −/− mice, there is decrease in the number of resting and proliferating chondrocytes; the columnar organization of the growth plate is disrupted, there is premature acceleration of chondrocyte maturation and apoptosis, and inappropriate ossification resulting in a dwarfing phenotype (a domed and foreshortened cranium, short limbs, small thorax) that is similar to that of Blomstrand chondrodysplasia (OMIM 215045), a disorder associated with loss-of-function mutations of PTH1R . Mice in which expression of Pthlh has been maintained only in chondrocytes survive but are short with cranial chondrodystrophy and failure of tooth eruption. In the heterozygous state ( Pthlh +/− ), mouse fetal development is normal but by 3 months of age, the trabeculae of the long bones are osteopenic; a similar bone phenotype is noted when loss of Pthlh expression is confined to osteoblasts. For comparison, in mice in which Pth has been “knocked out,” there is decreased mineralization of cartilage matrix, expression of vascular endothelial growth factor (VEGF) and neovascularization, osteoblast number, and trabecular bone volume. Thus both PTH and PTHrP are necessary for normal fetal endochondral bone development. Cortical thickness of long bones is increased in both Pth – and Pthlh -null mouse models, indicating that the regulation of endochondral and periosteal osteoblast function differs.
Serum concentrations of PTHrP are low except when it is secreted by tumors, leading to humoral hypercalcemia of cancer. There are high levels of PTHrP in breast milk and low concentrations of PTHrP may be measurable in the serum of lactating women.
Parathyroid Hormone/Parathyroid Hormone-Related Protein Receptors
PTH and PTH-rP bind to the PTH receptor, PTH1R. PTH1R is a 585 AA protein that is a member of the Class B family of GPCRs whose ligands include calcitonin, GH-releasing hormone, secretin, glucagon, glucagon-like peptide 1, gastric inhibiting polypeptide, vasoactive intestinal polypeptide, corticotropin-releasing hormone, and pituitary adenylate cyclase activating protein that are characterized by long extracellular amino-terminal domains (~ 160 AA residues) with multiple cysteine residues forming disulfide bridges. PTH1R has an extracellular domain, seven transmembrane spanning segments connected by three extracellular and three intracellular loops, and an intracellular domain with which the signaling G-protein interacts. PTH1R is composed of 15 exons; there are three promoters that regulate expression of PTH1R . As a result of alternative splicing of its mRNA, there are several isoforms of the PTH/PTHrP receptor.
The amino terminals of both PTH 1-34 and PTHrP 1-36 bind to the transmembrane and the extracellular loop sequences of the transmembrane segments of PTH1R, whereas their carboxyl terminals bind to the amino-terminal extracellular portion of PTH1R. Ligand-bound PTH1R transmits its message through several intracellular signal transduction pathways, including the G sα -adenylyl cyclase-cyclic AMP-PKA system, the G q/11 -PLCβ-inositol trisphosphate-cytoplasmic Ca 2 + -PKC path, the G 12/13 -phospholipase D-transforming protein RhoA system, and the β-arrestin-MAPK pathway. Interaction of the specific PTH1R ligand and PTH1R determines the signaling pathway and duration of response of these two components; thus PTH has a greater affinity for PTH1R than does PTHrP and coupling of PTH with PTH1R leads to a more stable, prolonged, and intense signaling response than does interaction of PTHrP and PTH1R. Indeed, even after internalization, PTH-PTH1R signaling continues.
After inducing intracellular signaling, PTH1R is phosphorylated by a GPCR kinase (GRK) and bound to β-arrestin-1 (encoded by ARBB21 ), leading to its internalization, and subsequent destruction or reutilization. Once internalized, PTH1R may be degraded, recycled to the cell membrane, or directed to the nucleus by importins-α 1 and -β where it is present in the nucleoplasm. The physiological functions of nuclear PTH1R and its ligands PTH and PTHrP are as yet unknown.
In the kidney, PTH1R is expressed on the apical and basolateral surfaces of cells in the proximal renal tubule; binding of PTH to apical PTH1R preferentially signals through cyclic AMP-PKA, whereas interaction of PTH with basolateral PTH1R activates the PLC-PKC signal transduction pathway. Through both PKA and PLC, PTH/PTH1R activates the MAPK signal transduction system of extracellular signal-regulated kinases (ERK)-1,2, allowing regulation of cell differentiation, division, growth, and apoptosis. In addition to participating in the internalization of the PTH-PTH1R complex, β-arrestin enables it to stimulate intracellular signaling in a manner that is independent of G-proteins; the GRK-mediated patterns of proline phosphorylation of PTH1R and β-arrestin likely determine their conformations and functional activities. Thus interaction of PTH-PTH1R with β-arrestin may also activate the MAPK signaling system and facilitate PI3K and PKB (AKT) intracellular signal transduction pathways. PTH-PTH1R activation of the MAPK pathway through its GPCR is rapid but relatively brief lasting only a few minutes, whereas activation through the β-arrestin pathway is slow and sustained over hours. Another mechanism through which PTH/PTH1R interaction affects cellular function is by the binding of PTH1R to the Na + -H + exchanger regulatory factor 2 (NHERF2—encoded by SLC9A3R2 ) with consequent stimulation of PLC activity and inhibition of adenylyl cyclase activity. In the kidney, PTH1R binds to NHERF1 (encoded by SLC9A3R1 ), an essential factor for trafficking of the phosphate channel NPT2a to the luminal membrane of cells in the proximal renal tubule. In the proximal renal tubule, linking of PTH to PTH1R increases cyclic AMP production, leading to decrease in the internalization and expression of the sodium-phosphate cotransporters and increase in urinary phosphate excretion; association of PTH1R with NHERF1 blunts the phosphaturic effect of PTH. Therefore disruption of PTH1R-NHERF1 interaction leads to renal wasting of phosphate. Agonists of PTH have been synthesized that can “bias” the selection of the signal transduction pathway used; a “biased” agonist might stabilize a receptor conformation that stimulates a specific signal transduction sequence or activate one pathway while simultaneously inhibiting another.
PTH1R is expressed in renal tubular cells and osteoblasts, skin, breast, heart, and pancreas among other tissues—the latter sites reflecting the paracrine targets of PTHrP. PTH, PTHrP, and calcitriol decrease expression of PTH1R . Targeted loss of PTH1R is accompanied by impaired proliferation of chondrocytes and acceleration of chondrocyte maturation and calcification, an outcome mimicked by targeted loss of G sα in chondrocytes. Clinically, inactivating mutations in PTH1R result in hypocalcemia and Blomstrand chondrodysplasia (OMIM 215045), whereas constitutively activating mutations of PTH1R lead to hypercalcemia and Jansen metaphyseal chondrodysplasia (OMIM 156400).
A second PTH receptor (PTH2R) is selectively activated by PTH but does not recognize PTHrP; PTH specificity is determined by Ile and Trp in native PTH, sites that affect activation and binding, respectively. PTH2R encodes a 539 AA GPCR with 50% homology to PTH1R that activates adenylyl cyclase; it is expressed predominantly in brain, testis, placenta, and pancreas, but not in bone or kidney; its physiological role is uncertain. In response to PTH 1-84 , PTH2R enhances both cyclic AMP generation and Ca 2 + mobilization. However, the naturally occurring endogenous ligand for PTH2R is not PTH but the 39 AA PTH-related hypothalamic tuberoinfundibular peptide (TIP39); this protein is also expressed in the testis and various central nervous system regions.
Although as yet not molecularly characterized, receptors for carboxyl-terminal fragments of PTH 1-84 have been functionally identified as renal and bone cell binding sites for PTH 1-84 from which intact hormone can be only partially displaced by PTH 1-34 but can be further displaced by PTH 53-84 and PTH 69-84 . 86 In addition, in osteocytes, osteoblasts, and chondrocytes from which the PTH/PTHrP receptor has been “knocked out” and to which intact PTH 1-84 but not PTH 1-34 binds, labeled PTH 1-84 can be displaced by carboxyl-terminal PTH 19-84, 28-84, 39-84 fragments. Important determinants for binding of PTH to the carboxyl-terminal selective receptor(s) appear to be AA 24 to 27 (Leu-Arg-Lys-Lys) and 53 to 54 (Lys-Lys). Furthermore, carboxyl-terminal fragments of PTH exert biological effects in intact and PTH1R -null cells, such as regulation of alkaline phosphatase activity in osteosarcoma cells and osteoblasts (but not generation of collagen type I), stimulation of Ca 2 + uptake by osteosarcoma cells and chondrocytes, and cell survival in vitro. Thus the physiological actions of PTH likely reflect the integrated sum of the individual functions of the intact hormone and its carboxyl-terminal fragments. Carboxyl-terminal fragments of PTHrP are not recognized by the sites that bind carboxyl-terminal fragments of PTH.
Calcitonin
Calcitonin, a 32 AA peptide encoded by CALCA , is synthesized and released by the neural crest-derived parafollicular C cells of the thyroid gland in response to rising concentrations of Ca 2 + (a reaction mediated by activation of the CaSR present on the plasma membrane of the C cell) and in response to several gastrointestinal hormones, including gastrin. CALCA is a six exon gene that by alternative transcription and translation forms a 141 AA protein from which calcitonin (exon 4) and katacalcin, a 21 AA hypocalcemic peptide adjacent to the carboxyl terminus of calcitonin, are derived, and a 128 AA protein from which is gleaned the 37 AA calcitonin gene-related-peptide (CGRP)-α (exon 5), a vasodilator and neurotransmitter that also interacts with the calcitonin receptor. Calcitonin lowers serum concentrations of calcium by inhibiting osteoclast-mediated bone resorption and by increasing renal calcium excretion. After binding of calcitonin to its GPCR expressed on the osteoclast cell membrane, the morphology of the ruffled membrane of the functioning osteoclast flattens causing it to withdraw from the bone reabsorption site, thereby decreasing this process and consequently lowering peripheral calcium concentrations. Calcitonin also decreases renal tubular reabsorption of filtered calcium. However, calcitonin enhances proximal renal tubular synthesis of calcitriol and thereby increases intestinal absorption of calcium. In turn, calcitriol suppresses synthesis and secretion of calcitonin; thus calcitriol has the same reciprocal relationship with calcitonin as it does with PTH and FGF23.
Calcitonin is produced in excess by medullary carcinoma of the thyroid and, at times, by other neuroendocrine tumors. The calcitonins of multiple species share similar structures including: five of the first seven amino-terminal AA, a disulfide bridge between AA 1 and 7, glycine at AA residue 28, and a proline amide residue at carboxyl-terminal AA 32. In the interior of the peptide, species other than human have several basic amino AA that make them more stable and easily recognized by the human calcitonin receptor and thus more biologically potent, for example, therapeutically useful salmon calcitonin.
Calcitonin secretion is stimulated primarily by increasing concentrations of Ca 2 + transduced by the CaSR expressed on the plasma membrane of the C cell. Members of the gastrin-cholecystokinin intestinal peptide hormone family (gastrin, glucagon, pancreozymin), glucocorticoids, and β adrenergic agonists are also potent calcitonin secretagogues. Calcium, pentagastrin, and glucagon are effective stimuli used to assess calcitonin secretion clinically. Somatostatin, calcitriol, and chromogranin A 1-40 inhibit and chromogranin A 403-428 stimulates calcitonin secretion. Calcitonin secretion declines as the Ca 2 + concentration declines. The half-life of calcitonin is brief; it is metabolized primarily by the kidney and also by liver, bone, and thyroid gland. Serum levels of calcitonin are high in the fetus (when serum total calcium concentrations are normally 12–13 mg/dL) and newborn, fall rapidly after birth as Ca 2 + values decline and then more slowly until 3 years of age remaining relatively constant thereafter (< 12 pg/mL). After 10 years of age, serum concentrations of calcitonin are higher in males than in females. The physiological role of calcitonin is unclear as serum calcium concentrations are normal in patients with both decreased (primary congenital or acquired hypothyroidism) and increased (medullary carcinoma of the thyroid) secretion of this peptide. However, disposal of a calcium load is slower in the calcitonin-deficient subject. Calcitonin values are slightly higher in males than in females. Immunoassayable concentrations of calcitonin are increased in patients with medullary carcinoma of the thyroid, chronic renal insufficiency, and pycnodysostosis.
The biological effects of calcitonin are mediated through its 490 AA class B GPCR encoded by CALCR . Intracellular signaling of the CALCR is transduced through the G sα adenylyl cyclase-cyclic AMP-PKA and G αq -PLC-IP 3 signal transduction pathways. Alternative splicing of CALCR results in two isoforms of the calcitonin receptor, one of which has an additional 16 AA inserted into its first intracellular loop between transdomains I and II. The calcitonin receptor is expressed in osteoclasts; when exposed to calcitonin, the osteoclast shrinks and bone resorbing activity declines quickly. Thus calcitonin lowers serum calcium and phosphate levels particularly in patients with hypercalcemia. In lactating women, serum levels of calcitonin rise and calcitonin is excreted in breast milk. Women who are breastfeeding their infants lose 5% to 10% of their trabecular bone mineral during 6 months of lactation that is recouped rapidly when lactation ceases, much more quickly than when bone mass is lost because of other problems (e.g., glucocorticoid excess, bed rest). Inasmuch as calcitonin values increase during pregnancy and lactation, calcitonin may play a role in the remineralization of bone in lactating women after completion of breastfeeding.
Vitamin D
Synthesis and Biological Activity of Vitamin D
Cholecalciferol (vitamin D 3 ) may either be generated endogenously by exposure of the skin to sunlight or consumed (in a diet consisting of oily fish, such as wild salmon, tuna, herring, cod, and mackerel; fungi, such as shiitake mushrooms; and egg yolks or as a dietary supplement). (Ergocalciferol [vitamin D 2 ] is a plant and yeast sterol that may either be consumed or ingested as a dietary supplement.) In the cutaneous stratae basale and spinosum, vitamin D 3 is synthesized from cholesterol: 7-dehydrocholesterol is photoisomerized to previtamin D 3 by ultraviolet B radiation at 290 to 315 nm and heat (37° C) from sunlight and then spontaneously isomerizes to cholecalciferol (vitamin D 3 ) ( Fig. 9.4 ). Previtamin D 3 is also metabolized into products, such as lumisterol 3 and tachysterol 3 and to inactive photoproducts when exposure to sunlight is excessive, thereby preventing vitamin D intoxication by exposure to sunlight alone. Cutaneously synthesized vitamin D 3 enters the circulation directly. Ergocalciferol (vitamin D 2 ) derived from ergosterol differs structurally from vitamin D 3 by the presence of a double bond between carbons 22 and 23 and a methyl group on carbon 24. Vitamins D 3 and D 2 exert reasonably similar biological effects when ingested in equivalent physiological doses; however, the biological potency of vitamin D 2 is less than that of vitamin D 3 because it is rapidly cleared from the circulation, restricting its further processing into a biologically active form. The latitude, season of the year, and time of day influence the rate of cutaneous synthesis of vitamin D 3 in response to exposure to sunlight; in higher latitudes, the path through which ultraviolet B radiation from the sun travels is longer and more of the ultraviolet radiation is absorbed by the ozone layer; thus less reaches the surface of the earth. Exposure of the back of a white adult to intense summer sun (mid-July) for 10 to 12 minutes in the northeastern United States generates around 10,000 to 20,000 IU of vitamin D 3 over the next 24-hour interval. (For persons of color, 30–120 minutes of exposure to sunlight may be required for comparable effects.) Sun-blocking topical creams and aging also decrease the cutaneous formation of cholecalciferol in response to sunlight. Both forms of vitamin D undergo similar chemical modifications to become bioactive metabolites. Orally ingested vitamin D is packaged into chylomicrons and absorbed into the intestinal lymphatic system and then enters the circulation. Vitamins D 3 and D 2 and their metabolites are transported in serum linked to vitamin D-binding protein (DBP), a polymorphic variant of the serum α2-globulin synthesized by the liver and encoded by GC . DBP binds vitamin D and its hydroxylated metabolites with high affinity and capacity.
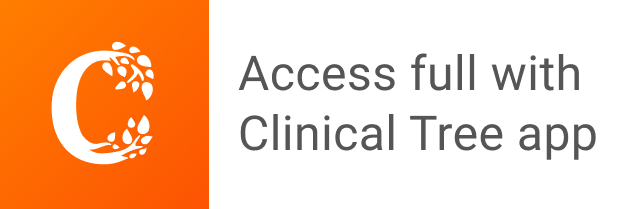