Fig. 14.1
Intraoperative ultrasound images of a 4-year-old boy with a low-grade glioma of the left frontal lobe. Preresection (a) and postresection (b) coronal plane images demonstrate the distinct echogenicity of neoplastic tissue (large arrow) from the surrounding brain. The midline falx cerebri is seen as an anatomic reference point (small arrows). (c) Doppler ultrasonography highlights the arterial blood supply of a choroid plexus papilloma
14.2.2 Neuronavigation
Conventional MRI provides the necessary detail to assess the anatomic relationships of most intracranial tumors. Several manufacturers supply systems that use a preoperative MRI scan as the basis for an intraoperative three-dimensional guidance system. In their various configurations, these are all considered neuronavigation systems. Standard MRI may be complemented by more specialized MR techniques (see Chap. 13) such as MR spectroscopy (MRS), diffusion tensor imaging (DTI), and functional MRI (fMRI), in addition to other tools such as magnetoencephalography (MEG) and conventional catheter angiography. These special MR sequences can be merged with conventional anatomic images and then used in the operating room during surgery.
Neuronavigation has impacted brain-tumor surgery in three major ways. First, surgical routes can be simulated and planned preoperatively, allowing maximum accuracy of craniotomy placements and cortical incisions. As a result, mistakes in trajectory and depth during tumor resection are prevented. Second, the shortest and safest route through brain tissue that avoids important neural and vascular structures can be determined well before the procedure. This reduces the risk of postoperative neurologic deficits, and improves assessment of risk preoperatively. Third, the use of neuronavigation improves the extent of tumor resection by providing “feedback” to augment the surgeon’s perception of anatomic placement. Despite all these advantages, the major limitation of preoperative registered imaging data is obvious: intraoperative shifts of tissue cannot update a static set of data obtained prior to tumor resection.
The original intraoperative navigation systems used metal stereotactic frames rigidly attached to the calvarium. These were referred to as “frame-based” stereotactic devices. Target guidance relied upon anatomic coordinates as defined in anatomic atlases. Coordinates and targets could be refined modestly by imaging studies such as plain films and air ventriculograms. The inherent inaccuracy of this system is obvious, in that patient-specific data is not used to guide the actual procedure. A key advance occurred when computed tomography or MRI scans were used in conjunction with frame-based systems (e.g., Leksell, Brown-Roberts-Wells [BRW], Cosman-Roberts-Wells [CRW)]) to select targets and mathematically compute trajectories. These systems use guidance arcs that move directly over the surgical field and obstruct the surgeon if a large craniotomy is to be performed. These systems are, however, highly accurate and continue to be used widely in situations where target selection is critical such as Gamma Knife radiosurgery and functional procedures such as pallidotomy (both of which utilize refinements of the Leksell frame, Elekta AB, Stockholm).
Advances in technology with sufficient computational power to allow manipulation and calculation of three-dimensional image sets represented a major innovation in the field. This led to the creation of systems known as “frameless stereotaxy” that recreated a three-dimensional volume space by using fiducials placed on the patient’s head and registered to a preoperative imaging study. This allows “real-time” intraoperative guidance and the facility to visualize the target in multiple planes (Fig. 14.2). A variety of tools, including surgical instruments, can also be registered and used as pointers that provide continuous updated information on a computer monitor as the tumor resection is performed. Many centers routinely use neuronavigation in all pediatric brain tumor cases. The problem of keeping scalp fiducials in place was solved by the use of surface registration of facial landmarks (Gleason et al. 1994).


Fig. 14.2
A screen capture image from a brainstem biopsy procedure. The biopsy trajectory is shown in three planes and the tip of the biopsy needle can be tracked in real time as it enters the brain (“Probes Eye” view, bottom right). StealthStation is a trademark of Medtronic Corporation (Minneapolis, MN)
Intraoperative MRI (iMRI), the next step in image-directed surgery, allows continual updating of preoperative image sets and adjustment of navigational parameters. This technique has been used to augment posterior fossa procedures (Fig. 14.3; Lam et al. 2001; Chen et al. 2007). Some reports indicate that the use of intraoperative imaging improves the extent of resection, although it is unclear whether it results in improved outcomes (Fahlbusch et al. 2000; Schneider et al. 2001; Avula et al. 2013). An intermediate step is the use of intraoperative-ultrasound-assisted navigation as a means to update imaging data (Regelsberger et al. 2000). Integration with functional information obtained from MRS, DTI, and fMRI allows intraoperative “guide-posts” for the surgeon, delineating regions amenable to resection and regions representing eloquent cortex or functional tracts (Nimsky et al. 2006; Gulati et al. 2009).


Fig. 14.3
A series of sagittal T2-weighted images from a 6-year-old girl with a grade II cerebellar astrocytoma. The first image (a) shows a poorly defined, cerebellar mass. After the initial resection during which the tumor margins were poorly defined, an intraoperative MR scan was done. It shows residual tumor at the base of the resection cavity (b). The patient was returned to the OR and a further resection was performed after which a final scan was done (c)
14.2.3 Functional Imaging
Several imaging technologies have been adapted to create functional maps of the brain: fMRI, diffusion weighted imaging (DWI), positron emission tomography (PET), and MEG. Differences that occur in blood flow between the active and inactive cortical areas are exploited by fMRI. These differences are magnified by instructing the patient to perform repetitive tasks, which may be as simple as repeatedly moving the fingers. Local increases in blood flow are then detected by specific MRI sequences. Patient cooperation is, of course, required.
DTI exploits the differences in the diffusion of water molecules depending on the local environment of those molecules (e.g., water molecules within axons vs. those in the interstitial space) (Pierpaoli et al. 1996). This information can then be extracted to create maps demonstrating the location and direction of white-matter pathways. Large white-matter pathways are particularly well-identified using these techniques (see Chap. 13, Fig. 13.7).
Preoperative use of PET was originally presented by LeBlanc and Meyer (1990) and Leblanc et al. (1992). PET relies on metabolic differences within active cortex to isolate functional areas. More recently, a variety of radiolabeled compounds such as [18F] fluorodeoxyglucose (FDG), [11C] l-methionine, and [15O] H2O were used by Kaplan et al. to create functional maps prior to brain tumor resection in a pediatric population (Kaplan et al. 1999). Coregistering [15O] H2O PET images with MRI allowed accurate determination of eloquent cortex prior to tumor resection. PET has also been used to select targets for biopsy within brainstem tumors (Pirotte et al. 2007).
MEG relies on the ability to detect single dipole magnetic fields created by the pooled activity of groups of neurons to define potential areas of seizure activity (Chuang et al. 1995; Otsubo et al. 2001). Further refinement of these techniques, known as magnetic source imaging (MSI), allows the identification of functional areas of cortex and deep brain regions (Fig. 14.4). This information is especially valuable when correlated with tumor localization (Schiffbauer et al. 2001). Delineation of entire functional pathways using a combination of techniques may be possible in the near future.


Fig. 14.4
Magnetic source imaging (MSI) of a 15-year-old boy with an infiltrative anterior insular mass (not visible in this image). Green squares demonstrate sensory cortex representing left fifth finger, right index finger, and right first finger. These functional maps were integrated into the neuronavigation system prior to tumor resection
14.2.4 Cortical Mapping for Supratentorial Tumors
The gold standard for functional mapping of the human brain is direct electrical stimulation of the cortex and observation of its effect upon patient-directed actions during open craniotomy (Berger and Ojemann 1992). Although pioneered in the early part of the twentieth century, continual refinements are improving the sensitivity and accuracy of these techniques. For the most part, the vast majority of mapping cases are restricted to either motor or speech mapping. The major limitations of cortical mapping in children are the relative immaturity of the central nervous (CNS) system in very young children and the inability of children to cooperate in the execution of repetitive language tasks during speech-mapping procedures.
Motor mapping as described by Penfield remains the most robust electrical technique that can be practiced in the operating room (Penfield and Boldrey 1937). Patients remain under general anesthesia and direct systematic electrical stimulation of the precentral area permits the accurate mapping of primary motor cortex. Areas of cortex responsible for specific muscle groups (e.g., face, arm, hand, leg) can be reliably identified. A bipolar electrode with 5-mm spacing is used to deliver stimuli at 60 Hz with duration of 1 ms (biphasic square wave pulse). In children less than 5 years of age, the cortex is generally less excitable by direct stimulation and motor cortex may not be clearly identified. An alternative method to detect the location of the central sulcus is by detecting a phase-reversal potential as one records over the motor and sensory cortex. Using subdural grids, mainly for the treatment of patients with epilepsy, Chitoku et al. were able to define the motor cortex in all children studied, using a variety of stimulation thresholds (Chitoku et al. 2001). Younger children responded to stimuli in the range of 8–12 mA, while older children responded to stimuli in the range of 4–6 mA.
For language mapping, a basic surgical decision point is whether a child can tolerate an “awake craniotomy” (usually above 10–12 years of age). Language mapping is entirely dependent upon patient cooperation and, therefore, is the most difficult technique to accomplish in young children. During an awake craniotomy, a handheld stimulator is used to directly inactivate the cortex, while the patient names objects presented visually. Stimulation of Broca’s area, which is often adjacent to the primary motor cortex, usually leads to speech arrest although significant variability exists between individuals of different ages (Haglund et al. 1994; Ojemann et al. 2003). Current techniques of speech mapping allow accurate intraoperative localization of speech function with minimal long-term impacts upon speech function following tumor resection (Sanai et al. 2008).
In children unable to tolerate an awake craniotomy, and for whom functional localization is crucial to the success of the procedure, placement of subdural grids allows bedside cortical mapping. This requires two procedures for the patient, a substantial degree of patient cooperation, and close communication between child neurologists, psychologists, and nursing staff. Generally, this technique can be performed in children above the age of 4 years, although it is most reliable in older children. The first procedure involves a craniotomy encompassing the area of resection and placement of an implantable subdural grid containing multiple electrical contacts. The contacts on the grid are then stimulated sequentially, while the patient is led through specific language tasks such as naming, counting, and repeating. As with awake craniotomy, speech arrest during stimulation is the clue for identifying active cortex. Language paradigms have to be tailored according to the age of the patient and level of comprehension. The procedure is time-consuming and laborious, often requiring sessions over 2 or 3 days. In the patient shown in Fig. 14.5, an infiltrative tumor was noted in the left frontoparietal region. Although the patient was but 5 years old, he tolerated placement of subdural grids for several days and bedside mapping revealed the locations of the language and motor cortex allowing an extensive resection of the tumor.


Fig. 14.5
An implanted subdural grid used to map speech and motor cortex in a 5-year-old boy with an infiltrative tumor of the left hemisphere. A 64-contact subdural grid was implanted over the area of the tumor (left image). Speech cortex was localized to numbers 2 and 3 during two sessions in the telemetry unit, and motor cortex to numbers 1, 4, 5, and 6 (right image). The location of the tumor was correlated with intraoperative neuronavigation and an extensive resection was performed
14.2.5 Mapping of Seizure Foci
If seizures are particularly intractable or appear to be the dominant symptom associated with a brain tumor, the actual ictal focus may need to be identified. For most types of epilepsy associated with a definite lesion, resection of the lesion will result in seizure control in the majority of cases (Mosewich et al. 2000). In some cases, the presence of a tumor is only detected after pathologic examination of the resected tissue. In a series of surgical treatment for epilepsy, approximately 25 % of temporal lobe resections revealed a neoplasm, the majority of which were dysembryoplastic neuroepithelial tumors (DNET, see Chap. 8) (Hennessy et al. 2001).
Scalp electroencephalography is an essential first step in localization of seizure foci. This may be complemented by MEG or invasive monitoring with subdural grids and strip electrodes. Insertion of a subdural grid electrode array provides ictal and interictal information. Strip electrodes are used for recordings from mediobasal structures. In addition, recording along the hippocampus can be performed following the removal of lateral temporal cortex and entry into the temporal horn of the lateral ventricle. Strip electrodes may also be used for the orbitofrontal cortex or under the bone flap, if the cortical exposure is not adequate. The recording may either be done for short time periods intraoperatively (5–20 min) prior to resection, or for prolonged periods postoperatively in the ward in specialized monitoring units. Intravenous infusion of methohexital (Brevital 0.5–1 mg/kg) may be used to chemically induce ictal discharges if epileptiform activity is sparse. Following tumor removal, electrocorticography is always performed in patients with identifiable preresection seizure foci. Infrequent spike activity is not pursued, especially when it involves functional cortex. Resected seizure foci are identified with respect to their geographic orientation to the tumor nidus and should be submitted separately for histopathologic analysis.
14.2.6 Laser Ablation
A relatively new addition to the array of surgical techniques available for the management of CNS tumors is focused laser thermal ablation (Visualase, Medtronic Inc, Houston, TX; Carpentier et al. 2012; Hawasli et al. 2013). In comparison to open surgery, where the goals are exposure, visualization of tumor margins, and dissection of tissue planes, laser ablation therapy targets neoplastic tissue in situ through a minimally invasive approach. First, the target is identified on preoperative MR imaging. Next, a laser fiber is delivered to the target using stereotactic guidance. Surgeons have the option of using frame-based coordinates or frameless navigation to implant the laser, then confirm accurate placement with an MRI. The fibers are held in place with a small skull anchor and require a small skin incision and minimal hair removal (Fig. 14.6a). Alternatively, lasers can be delivered under direct visualization in an iMRI suite. This is most easily achieved with a skull mounted Smartframe and adjusted in real time with the guidance of Surgivision ClearPoint implantation software (MRI Interventions, Irvine, CA; Fig. 14.5b). The progress of laser therapy can then be monitored both with MR-thermography and with software that estimates the volume and extent tissue ablated (Fig. 14.6c).


Fig. 14.6
Fixation devices for implanted laser ablation fibers. (a) Skull anchors for laser fixation can be placed using frame-based or frameless techniques and do not require large incisions or hair shaves. (b) The ClearPoint SmartFrame can be mounted above a burr-hole whose position is optimized with trajectory software. (c) The extent of ablation can be estimated with thermography and software that calculates tissue damage
14.3 Posterior Fossa Tumors
14.3.1 Surgical Principles
Posterior fossa tumors are among the most commonly encountered tumors in children and are located in the midline within the vermis, cerebellar hemisphere, and/or brainstem. Standard approaches to the posterior fossa are well developed and are described in various sources in the literature. Surgical planning is often modified by the pathology of the tumor. The three main pathologic types that occur in this location are astrocytoma, medulloblastoma, and ependymoma. Cerebellar astrocytomas are usually eccentric in location, associated with a cyst, and often cause obstructive hydrocephalus. Medulloblastomas are characteristically midline tumors occupying the vermis and in some cases extending into the cerebellar hemisphere. Ependymomas of the posterior fossa can extend into the fourth ventricle and also may extend into the cerebellopontine angle closely associated with the cranial nerves. Both ependymoma and medulloblastoma can invade the brainstem.
For midline tumors, the standard approach is to position patients prone, with the head flexed forward. A vertical incision allows access to most of the posterior fossa. If the tumor extends anterior to the cerebellum or brainstem, the tumor must be approached from a more lateral position, often with the head turned to allow a posterolateral trajectory. In the past, most surgeons would remove the overlying bone without replacement (craniectomy), but now, most surgeons attempt to replace the bone flap at the conclusion of the procedure (craniotomy). There is some evidence that this reduces the risk of cerebrospinal fluid (CSF) leakage and pseudomeningocele formation (Gnanalingham et al. 2002). Dural opening is followed by definition of the boundary between the tumor and the normal cerebellum. The character of the interface between tumor and brain can vary across tumor types. In general, the interface is better defined with ependymomas as compared to medulloblastomas. Cerebellar astrocytomas have a clear margin between the tumor and the adjacent brain. The large size of most pediatric tumors and the need to avoid unnecessary brain retraction results in many tumors being resected piecemeal using either standard tools (cautery and suction) or ultrasonic aspirators that use a rapidly vibrating metal tip to disintegrate tissues. Removal of the central portion of the tumor then allows reflection of the deeper tumor tissue into the operative field and subsequent removal. For deep tumors, and those related to important structures, the use of frameless stereotaxy (neuronavigation) has revolutionized intraoperative guidance and structure localization.
Finally, additional safety can be obtained by monitoring various cranial nerves such as the facial, oculomotor, and hypoglossal nerves (Grabb et al. 1997; Sekiya et al. 2000). Tumors either infiltrating the floor of the fourth ventricle or along the anterior portion of the brainstem involve cranial nerves. These nerves are sensitive to manipulation and can be difficult to identify when tumors surround them. Gradual dissection through tumor tissue requires careful identification of important structures.
14.3.2 Complications
Surgical resection of posterior fossa tumors may lead to both nonneurologic and neurologic complications (Table 14.1). Fortunately, the majority of these adverse effects are either transient or treatable, making the overall morbidity quite low. The tendency of posterior fossa tumors to occupy a midline position creates a stereotypical pattern of symptoms following surgery.
Table 14.1
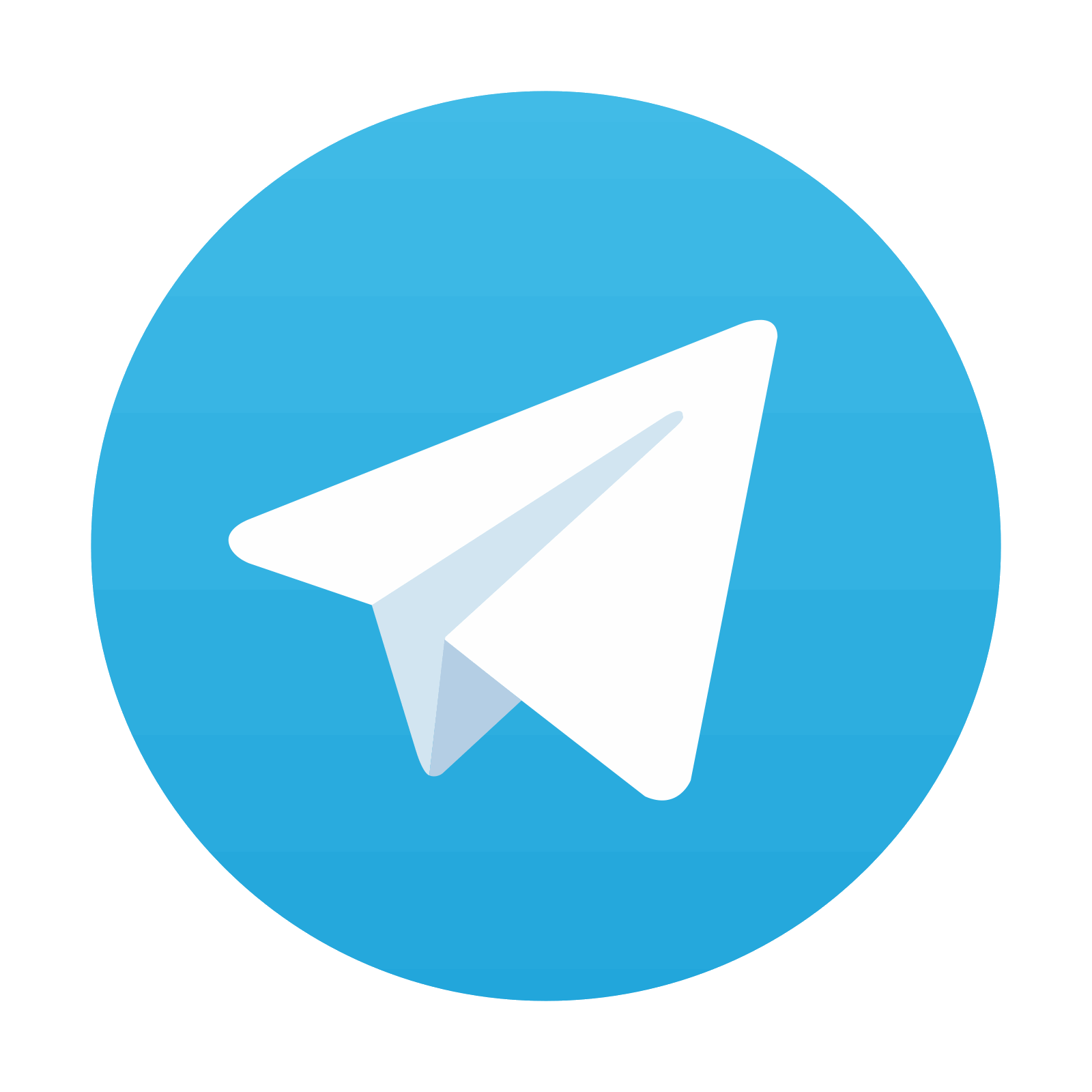
Complications following surgery for cerebellar astrocytoma
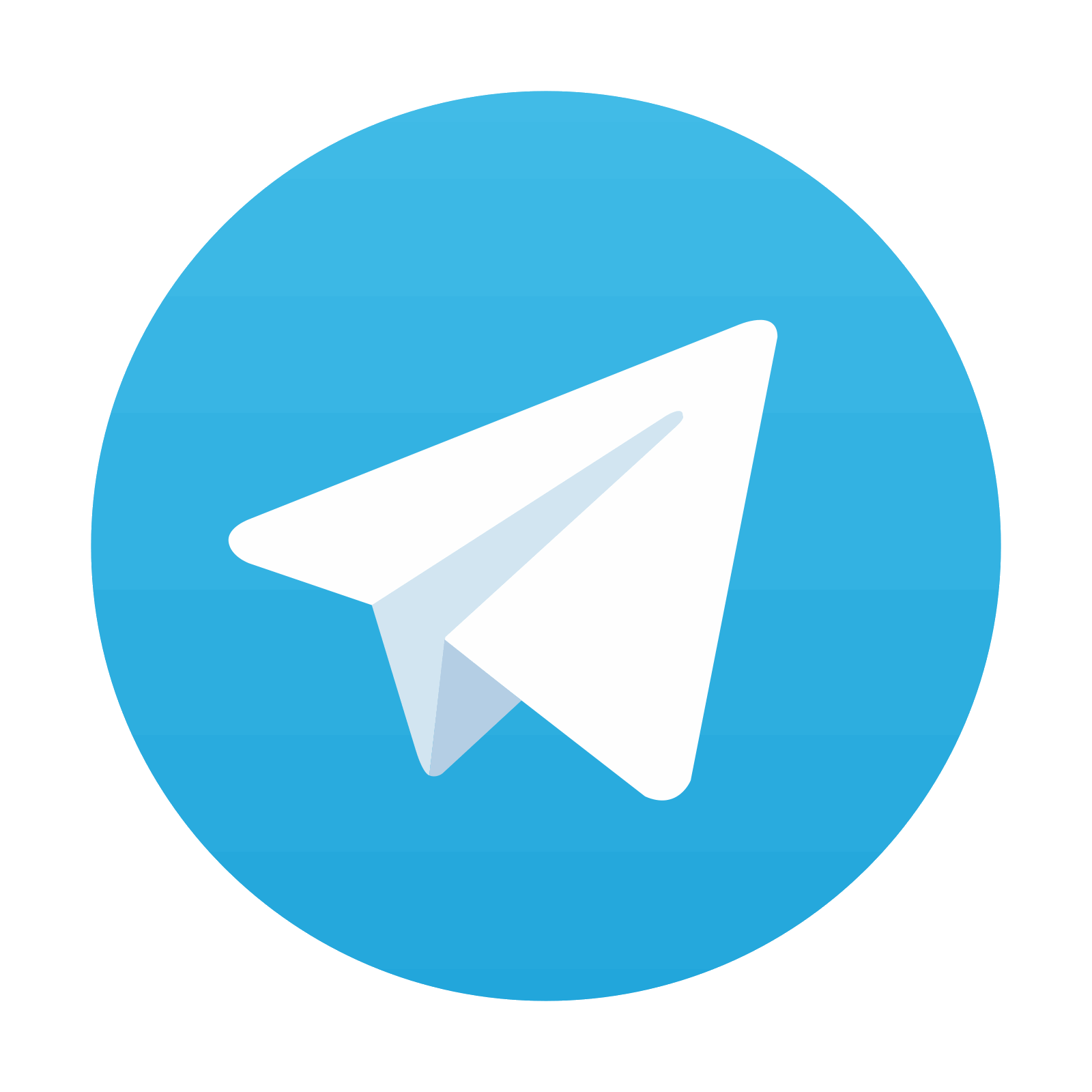
Stay updated, free articles. Join our Telegram channel
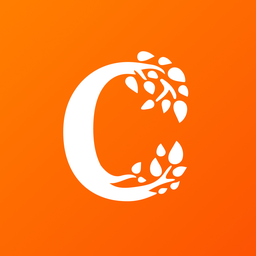
Full access? Get Clinical Tree
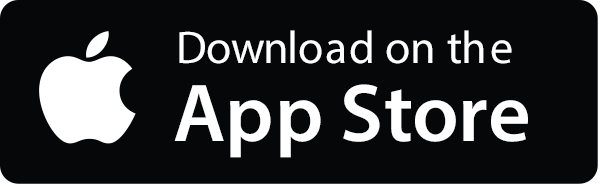
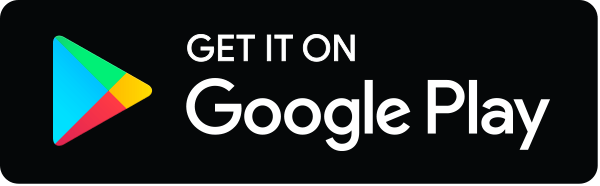
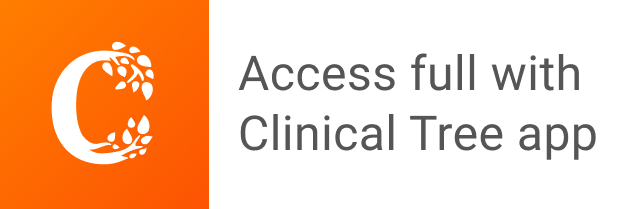