Fig. 4.1
Early chromosome analysis and molecular cytogenetics. (a) Photograph of Theodor Boveri (1862–1915). Boveri was a professor of zoology in Würzburg, Germany. Combining his theory that chromosomes carry genetic information with observations he made when studying cell division in ascaris larvae and double fertilized sea urchin eggs, Boveri postulated that cancer is a disease of the chromosomes. These studies were published in 1914 with the title “Zur Frage der Entstehung maligner Tumoren.” (b) Cover of the English edition of Boveri’s book, which appeared in 1929. His wife and former student Marcella Boveri translated the text. (c) Additional centrosomes and aberrant mitoses in ascaris. These observations led Boveri to conclude that tumorigenesis ensues as a consequence of the loss of inhibiting and the gain of promoting chromosomes. (d) SKY analysis of chromosomes from a patient with chronic myelogenous leukemia. The balanced translocation between chromosomes 9 and 22, t(9;22), the so-called Philadelphia chromosome, is readily visible (arrowheads). The consequence of this translocation is the generation of a fusion protein with increased tyrosine kinase activity, generated by the juxtaposition of the genes BCR and ABL. Note that all other chromosomes are normal. (e) G-banded, normal male human karyotype. Chromosome banding revolutionized the discipline of cancer cytogenetics by providing the possibility to identify nonrandom, cancer specific chromosomal aberrations. (f) Dual color hybridization targeting chromosome 8 (red) and the MYC oncogene (green), which resides on chromosome band 8q24. Note that chromosomes occupy specific territories in the interphase nucleus. The MYC oncogene, expressed in the colon cancer cell line DLD1, is contained in the territory. Note that most of the territories are in the periphery of the nucleus, consistent with the fact that chromosome 8 is gene poor. (g) Dual color high-resolution mapping of multiple BAC clones on the long arm of chromosome 6. These clones are part of the CCAP collection, which was established to systematically integrate the cytogenetic and sequence maps of the human genome by FISH mapping sequenced BAC clones (http://cgap.nci.nih.gov/Chromosomes/CCAP). (h) Chromogenic in situ hybridization (CISH) of a breast cancer sample with probes detecting the ERBB2 oncogene. The brown precipitates indicate high-level amplification in cancer cells. The adjacent lymphocytes are negative. The slide was stained with hematoxylin (blue). (i) Silver-enhanced in situ hybridization (SISH) of a malignant brain tumor (glioblastoma multiforme) showing amplification of the gene encoding the epithelial growth factor receptor, EGFR. The pronounced black stain can be analyzed automatically. Cells of vascular proliferation (right side of the slide) are negative for this gene amplification. The slide was stained with hematoxylin (blue).
Progress in the years to follow was slow. This was probably not attributable to a lack of interest or due to an a priori rejection of Boveri’s hypotheses , but due to insurmountable challenges in the analysis of chromosomes. The analysis of tissue sections per se was exceedingly difficult, fixation and preparation techniques were insufficient, and the preparation of metaphases slides was impossible, to name just a few hurdles for a systematic cytogenetic approach to the analysis of chromosomal abnormalities. The inability to identify consistent cancer-specific cytogenetic changes did not help to promote the field, and it is very conceivable that the subsequent frustration, which was mainly attributable to shortcomings in analytical technologies , supported the prevalent interpretation that cytogenetic abnormalities were present in cancer, but were merely disease-correlated , but not the cause of this disease [9, 10]. The fact that the correct number of human chromosomes was only established 4 years after the structure of the double helix was deciphered attests to the problems that cytogeneticists faced [11, 12] (the reader may consult the enlightening publication by Kottler, who discussed some additional shortcomings in cytogenetics at that time [13]).
After establishment of the normal human chromosome count, the field of human cytogenetics exploded. In particular, numerical chromosomal aberrations associated with congenital abnormalities were reported in short order [14–18]. However, the identification of recurrent aberrations in cancer cells came later. In 1960, Nowell and Hungerford described a consistent marker chromosome (termed the Philadelphia chromosome) in patients with chronic myelogenous leukemia (CML) [19]. This description was undeniably facilitated by the discovery that lymphocyte cultures can be stimulated with phytohemagglutinin (PHA) by the same authors [20]. For the first time, cancer was associated with a specific genetic abnormality that is visible through a microscope. The importance of this discovery cannot be overestimated. The consistency with which the Philadelphia chromosome was observed in CML all but proved that its presence reflected the molecular lesion accounting for the development of this hematological malignancy . Yet technical hurdles again prevented a thorough characterization of this marker chromosome because the structural integrity of chromosomes could not be analyzed in detail due to the lack of discernible features along individual chromosomes. This changed with the introduction of chromosome banding techniques [21, 22] (Fig. 4.1d). This revolution also facilitated the identification of intrachromosomal changes, and most importantly, the description of the reciprocal character of the Philadelphia chromosome—a balanced translocation involving chromosomes 9 and 22 [23] (Fig. 4.1e). Many more specific reciprocal translocation were subsequently identified, the translocation t(8;14) in Burkitt’s lymphoma among them [24, 25]. Similar consistent aberrations were found in mouse models of human cancer, such as those characterized in murine plasma cell tumors [26, 27]. Together, these findings prompted the development of the hypothesis that the sites of the consistently observed breakpoints pointed to the location of cancer-promoting genes, which would be activated as a consequence of such translocations [28]. In fact, this was the case. The human MYC oncogene is juxtaposed to the immunoglobulin heavy chain gene via the translocation t(8;14) in Burkitt’s lymphom a [29, 30], and an equivalent situation occurs in mouse plasma cell tumors on the respective orthologous chromosomes [26, 31]. The functional consequence is the deregulation of MYC expression by an active promoter of IGH in hematological cells, which is sufficient for malignant transformation. The Philadelphia chromosome represents a second mechanism for the translocation-induced activation of an oncogene —the generation of a fusion transcript with constitutively active tyrosine kinase—again sufficient and the cause for the emergence of leukemic cells.
The paradigm of translocation-induced oncogene activation and tumorigenesis was discovered based on cytogenetic analyses [32]. The importance of these discoveries cannot be overstated—not only does the detection of such aberrations serve as a diagnostic and prognostic tool and can be used for monitoring the efficacy of treatments, but it also identifies specific molecular targets for therapeutic intervention. For instance, BCR-ABL is the target for Gleevec in the case of CML [33]. The comprehensive analysis of chromosomal aberrations in solid cancers remained more complicated and had to await the development of molecular cytogenetic techniques that we review in the following sections.
4.2 The Emergence of Molecular Cytogenetics
The development of chromosome banding techniques is likely the single most important technical development in cytogenetics. Chromosome banding was first developed by Torbjörn Caspersson and Lore Zech in Stockholm using quinacrine mustard fluorescence and was immediately applied to establish karyotypes of hamster, human, and mouse chromosomes. As described above, the application for the detection of chromosomal abnormalities followed in short sequence. A conference held in Paris in 1971 published an official version of the human karyogram and established a map of human chromosomes. With the introduction of Giemsa banding by Seabright in 1971 [34], which omitted the requirement for fluorescence microscopy and generated permanent banding patterns, the method quickly entered laboratories around the world, and for decades to come was the most frequently performed genetic test (Fig. 4.1d). Besides its application in cancer, chromosome banding has become the method of choice for the detection of constitutional chromosomal abnormalities , both in the postnatal and prenatal settings. While the knowledge gained through the systematic application of chromosome banding techniques is vast, the technique has limitations. First, the resolution is low. Second, chromosome banding can only be applied to fresh tissues from which the cells can be cultured and arrested in metaphase (or prometaphase when higher resolution was desired). Third, the interpretation of banded karyotypes requires a skill set that not everyone can muster. These limitations combine to account for the fact that considerably more karyotypes from hematological malignancies have been published than from solid cancers [35, 36]. Most solid cancers are difficult to culture, and the morphology of metaphase chromosomes is often poor. In addition, the sometimes enormous number of cytogenetic abnormalities in solid cancers prevented a comprehensive analysis of all aberrations, and cytogenetic correlates of oncogene amplification, such as double minute chromosomes (dmin) and homogeneously staining regions (hsr), could be described, yet their chromosome of origin remained elusive. Most of these shortcomings were overcome by the marriage of classical cytogenetic chromosome analysis with independently developed molecular techniques (DNA cloning and hybridization).
4.2.1 In Situ Hybridization
The first relevant achievement was the successful in situ hybridization with radioactively labeled nucleic acids by Joe Gall and Mary Pardue in 1969 [37–39]. This was followed by the first in situ hybridization with fluorescently tagged probes by Rudkin and Stollar in 1977 [40]. Clearly, omitting radioactivity had intrinsic advantages, such as increased resolution, shorter detection times, and the possibility to increase the number of simultaneously detectable targets. Rudkin and Stollar used indirect immunofluorescence for the visualization of RNA/DNA hybrids. Another very important development for the success of molecular cytogenetics was the development of covalent labeling of nucleotides with haptens, the first of which, and still one of the most widely used, was biotin [41]. These hapten-conjugated nucleotide analogs can be incorporated enzymatically into DNA molecules, hybridized, and visualized with suitable detection systems, such as avidin conjugated fluorochromes or antibodies against other haptens, such as digoxygenin or dinitrophenol. Their advantage lies in the fact that their use increases the multiplicity and sensitivity of in situ hybridization experiments, increasing experimental versatility. More recently, nucleotide analogs directly coupled with fluorochromes have become available as well. The authors of this chapter purposely abstain from providing details of protocols for in situ hybridization experiments. Such protocols can be found in great detail and abundance for instance in the following references [42–45] and websites (http://www.riedlab.nci.nih.gov/).
Using a hybridization format for cytogenetic analyses obviously brought with it the possibility of designing DNA probes that were suitable to answer the specific experimental question asked. Among the first probes used for fluorescence in situ hybridization (FISH) were probes that targeted the centromeric or paracentromeric heterochromatin of specific chromosomes. Such probes readily allowed chromosome enumeration in metaphase preparations, but even more importantly enabled the concept of interphase cytogenetics. This meant that numerical chromosomal aberrations could be detected in nondividing cells, throughout all stages of the cell cycle, opening the door for pathologists to use cytogenetics to augment the morphology-based diagnosis of samples in the clinical laboratory. The concept and the term interphase cytogenetics were first conceived and published in a paper by Cremer and colleagues, who used a radioactively and nonradioactively labeled probe to detect the number of chromosome 18 in fibroblasts [46]. The concept of interphase cytogenetics proved to be very useful for the detection of aneuploidies in prenatal diagnostics, where numerical chromosomal abnormalities of chromosomes 13, 18, and 21 play a major role for constitutional syndromes, which previously could be diagnosed only after in vitro culture of amniotic fluid cells [47]. Interphase FISH is now widely used in the screening for chromosomal disorders in prenatal diagnostics.
4.2.1.1 Chromosome Painting
A second set of probes that became immensely useful in the diagnosis of chromosomal disorders were probes that allowed delineation of entire chromosomes. Chromosome painting probes were first reported for the human Y chromosome by Christoph Cremer, Joe Gray, and colleagues at the University of Freiburg and the Los Alamos National Laboratory [48]. Two groups, one with Thomas Cremer and David Ward at Yale University, and the other with Dan Pinkel and Joe Gray at the University of California in San Francisco, developed methods for painting specific chromosomes based on suppression hybridization [49–51]. Initially, such painting probes were cloned in phage or plasmid vectors [52], which resulted in acceptable signal to noise ratios. However, chromosome painting probes were soon prepared by either flow-sorting or by microdissection of individual chromosomes [53, 54]. Both techniques require amplification of the isolated nucleic acids using universal DNA amplification [55], but the signal to noise ratio is superior to that of the cloned chromosome libraries. Suppression of repetitive sequences is necessary to assure chromosome specific hybridization, which is achieved by including unlabeled DNA (either whole genomic DNA or repetitive sequence enriched Cot1-DNA) in the hybridization mixture. Chromosome painting probes are now routinely used to verify suspected aberrations in the clinical cytogenetic or in the cancer cytogenetic laboratory. Probes already labeled with different fluorescent dyes are commercially available from a variety of commercial suppliers. Chromosome painting probes have proven to be valuable experimental tools above and beyond their applications in diagnostics, for instance in the study of the three dimensional (3D) architecture of the interphase nucleus [56]. FISH with painting probes to interphase nuclei has unambiguously confirmed classical studies using UV-microbeam experiments [57] showing that chromosomes maintain their integrity throughout all stages of the cell cycle and are arranged as domains, or territories. Now we also know that chromosomes are not randomly distributed in the 3D nuclear space. Several groups have generated solid evidence that gene-rich chromosomes tend to be found more towards the interior compartment, whereas gene-poor chromosomes are positioned more towards the nuclear periphery [58, 59]. This organization appears to also be maintained to a certain degree in cancer cells, and in cells which contain artificially introduced chromosomes [60–62]. Chromosome painting has therefore become instrumental in attempts to decipher how the architecture of the nucleus affects transcriptional regulation in physiological states as well as in cancer, and how the dedifferentiation of cancer cells affects or is affected by the organization of chromosome territories. In combination with probes for specific genes, chromosome painting probes can be used to investigate whether the transcriptional state of a specific gene is reflected by the relative position in its territory [63, 64] (Fig. 4.1f).
4.2.1.2 Gene-Specific Probes
With the improvements in microscopic techniques and the availability of more and better probe labeling methods and fluorochromes, the versatility of FISH experiments also greatly increased [65]. These developments were paralleled by progress of the Genome Project and the availability of bacterial artificial chromosome (BAC) clones for essentially every gene. Historically, some of the first gene specific probes were cloned into lambda phage vectors and used for the visualization of specific chromosomal breakpoints associated with different malignancies, including CML and Burkitt’s lymphoma . For instance, the groups of Pinkel and Gray first succeeded in visualizing the BCR-ABL fusion in malignant cells from a patient with CML in interphase cells using a two-color hybridization format which resulted in a co-localization event on metaphase chromosomes and in interphase nuclei in which the Philadelphia chromosome was present [66]. Ried and colleagues developed a three-color approach that included a chromosome 8 painting probe together with probes specific for the MYC oncogene and probes for the IGH locus to achieve specific metaphase and interphase detection of the translocation t(8;14) in cell lines derived from a patient with Burkitt’s lymphoma [67]. The usefulness of this probe set was also explored for the detection of minimal residual disease [68]. The obvious advantage of being able to visualize specific chromosomal translocations directly in nondividing cells led the community of hematological oncologists to embrace the use of FISH very quickly. Today, specific FISH probes for a plethora of chromosomal translocations, inversions, deletions, and amplifications are available from a variety of suppliers. An appealing feature of FISH is that it can be combined with immunohistochemistry or specific pertinent stains. This allows identification of cancer-causing chromosomal aberrations in tissues that maintain the phenotypical appearance that a diagnostic pathologists is used to [69–71].
FISH probes are not restricted to specific genes known to be the target of chromosomal aberrations. The National Cancer Institute has established a resource that systematically integrates the cytogenetic and sequence maps of the human genome. This was achieved by mapping a set of more than 1300 sequence-verified BAC clones to high-resolution banded chromosomes. Any suspected cytogenetic abnormality can now be confirmed and characterized with these tools, and the molecular events at sites of chromosomal aberrations pursued to the very sequence [72–75] (Fig. 4.1g).
4.2.1.3 Molecular Cytogenetics for the Pathologist
While many molecular techniques never became part of routine pathology, FISH was implemented relatively soon after its development. This was due to the fact that the protocol was fairly simple, that tissue morphology was conserved, the method was affordable, and it was able to detect aneuploidy and structural chromosomal rearrangements , both highly relevant for carcinogenesis. Applying in situ hybridization with appropriate probes augments the morphological assessment and can contribute to diagnosis, differential diagnosis, and to prognostication. Using sequence-specific probes, virtually all known translocations can be identified. Next, we review two of the main nonfluorescent in situ hybridization formats used in routine pathology laboratories. In subsequent sections we summarize some of the major applications of in situ hybridization in pathology, with an emphasis on solid cancers.
4.2.1.4 Chromogenic In Situ Hybridization
Evaluation of FISH slides has some drawbacks since it requires an epifluorescence microscope, the analysis needs to be performed in a dark environment, and the signals eventually fade. To overcome these disadvantages, an alternative has been developed—chromogenic in situ hybridization (CISH) . CISH is based on the same molecular principles as FISH but uses chromogens instead of fluorescent dyes to visualize the hybridized probes. A comparison of features between FISH and CISH can be found in Table 4.1. The best staining results are obtained with indirect labelling protocols using either biotin or digoxigenin as haptens. The digoxigenin-labeled DNA probe is detected by using anti-digoxigenin antibodies, while the biotin labeled probe is visualized with avidin conjugated with enzymes such as alkaline phosphatase (AP) or horseradish peroxidase (HRP). These enzymes produce, in a secondary reaction, a stable precipitate of chromogens that are generated by the enzymatic conversion from soluble states. The sections are subsequently counterstained with hematoxylin and can be evaluated with bright field microscopy. Most of the current CISH applications are based on a single-color detection method, which does not allow simultaneous illustration of a reference probe on the same slide [76–78]. This limitation was overcome by the development of dual-color and triple-color in situ hybridization protocols [79–81]. Recent publications attest to the robustness and reproducibility of such protocols. The concordance with FISH based experiments is excellent [82, 83] (Fig. 4.1h).
Table 4.1
Overview of commonly used in situ hybridization systems in the pathology laboratory
FISH | CISH | SISH | |
---|---|---|---|
Automation | Semi | Semi | Full |
Specific equipment | Yes (epifluorescence microscope) | No (standard bright field microscopy) | No (standard bright field microscopy) |
Assay time | Overnight | Overnight | 6 h |
Scoring time | Time consuming, needs trained personnel and expertise | Fast interpretation of the results | Fast interpretation of the results |
Morphology conservation | Reduced due to fluorescence | Normal “IHC” morphology | Normal “IHC” morphology |
Signal quality | Good | Sometimes faint | good |
Multiple probes simultaneously | Yes, direct | Yes, indirect | Not yet |
Concordance with FISH | – | Good | Good |
Image Analysis | Yes, but not routinely | Yes, but not routinely | Yes, with fast results |
Processing time | 1 day | 0.5 days | 1 h |
Archivability | No | Long term | Long term |
4.2.1.5 Silver-Enhanced In Situ Hybridization
CISH overcomes some of the limitations of FISH, but has not yet found broad acceptance in pathology laboratories. The reasons for this might be the requirement for manual processing, overnight hybridization, and the need for two separate detection steps (one for the gene of interest and one for the reference probe). Furthermore, some CISH samples are difficult to evaluate because the signal can be weak. This led to the development of a fully automated in situ hybridization protocol, producing a stable and discrete chromogenic reaction product—silver in situ hybridization (SISH) [83]. SISH is fast and the experiment is analyzed by standard light microscopy. The technology applies an enzyme-linked probe, which in turn accumulates silver particles, forming a dense, punctuated, high-resolution black signal, which is easily recognizable. It provides a permanent record, with no fading or photobleaching, and its sensitivity allows visualization of single genes. The SISH technique is performed as an automated protocol and completed within one working day (about 6 h). Several studies have verified a very high concordance rate between SISH and FISH, proving clinical applicability [84–88] (Fig. 4.1i). A comparison of features between FISH and SISH can be found in Table 4.1.
4.2.1.6 Hybridization With Genomes
Chromosome banding, albeit with limited resolution, allows the survey of an entire genome. This feature is critical if the investigator has no a priori knowledge of the aberrations to be identified or characterized. The previously described molecular cytogenetic methods lack this characteristic and were therefore most useful to confirm, specifically and in nondividing cells or tissue sections, the presence of suspected aberrations. The research community therefore attempted to combine the advantages of both and developed genome-screening tools based on fluorescence in situ hybridization.
4.2.1.7 Comparative Genomic Hybridization (CGH)
The first such concept was realized with the introduction of comparative genomic hybridization (CGH) by Kallioniemi and colleagues at the University of San Francisco in California and by the group of Thomas Cremer in Heidelberg. Using a CCD camera (or other digital imaging device) fluorescent intensities can be measured quantitatively, that is the measured intensity is proportional to the amount of hybridized fluorescently labeled probe. If sequences in a test genome labeled with a green fluorochrome are more abundant than in a red-labeled reference genome, this difference should become visible upon hybridization to normal metaphase chromosomes as reflected by increased fluorescent signal. Conversely, losses of genetic material in the test genome would result in reduced green intensity. In papers published in 1992 and 1993 both groups presented convincing proof-of-principle experiments [89–91] by mapping genes amplified in cell lines and primary cancer, and by showing that by hybridizing DNA from individuals with constitutional chromosomal abnormalities such numerical imbalances can be identified. CGH has become one of the most important techniques in the study of cancer genomes, and essentially all major cancers and cell lines derived from them have been studied in great detail. Several databases now contain comprehensive catalogues of chromosomal imbalances, and several reviews summarize the many primary publications [92–94].
As apparent from the experimental concept, screening the genome for imbalances using CGH does not require the preparation of metaphase chromosomes from cancer samples for banding analysis. This feature was particularly useful for the analysis of solid cancers of epithelial origin (carcinomas), because cell culture of such samples is often difficult, and only for some carcinomas could cell lines be established in sufficient quantities. Karyotyping was further impeded because the morphology of metaphase chromosomes was poor, and in general the karyotypes revealed more aberrations compared to spreads prepared from hematological malignancies [36]. The fact that only cancer DNA was required for genome screening had another significant advantage—it opened the door to apply cytogenetic analysis to formalin fixed and paraffin embedded samples [95–97]. This feature allowed accessing the richly stocked pathology archives to perform retrospective analyses and to compare genomic aberration profiles with clinically relevant parameters, such as outcome and response to therapy. Moreover, it greatly facilitated the analyses of premalignant lesions. It is eminently difficult to establish cell lines for cytogenetic analysis from bona fide precursor lesions of carcinomas, and the earlier the lesions are situated in the sequential progression events to malignant, invasive disease the more normal cells are present. This problem could be overcome by dissecting morphologically identified premalignant lesions from surrounding normal tissue and extracting DNA exclusively from morphologically suspicious lesions [98, 99]. Of course, the earlier such lesions are detected the smaller they usually are, and not always is the quantity of DNA recovered sufficient for standard CGH experiments (which is in the range of a few micrograms). This limitation was overcome by the development of protocols for the sequence independent universal amplification of DNA using degenerate primers followed by cycles of PCR [100]. In essence, these combinations of methodologies enabled characterization of the sequential genomic imbalances in the progression of solid cancers. When combined with micro-dissection or macro-dissection of morphologically characterized lesions one could then establish a phenotype/genotype correlation and provide insights into the dynamics of genomic aberrations during tumorigenesis [99, 101]. Through improvements in protocols for microdissection, genome amplification, and CGH, ) genomic aberrations profiles could be established from only a few, and possibly from single cells, with considerable implications for the study of cancer heterogeneity, clonal composition, tumor dormancy, identification of disseminated cancer cells, and for the identification of the cell of origin of metastatic lesions [102–105].
CGH does not require the analysis of aberrant chromosomes, yet even the correct identification of DAPI banded normal chromosomes requires a certain cytogenetic skill set and prevented automation and high-throughput. Furthermore, the resolution with which chromosomal gains and losses could be detected is limited by the resolution of the target metaphase chromosomes (in the megabase range), and was dependent on the copy number change. High-level copy number amplifications could be more readily detected than subtle deletions (but the mapping resolution along the axis of chromosomes of course remained the same). Accordingly, the molecular cytogenetic community quickly realized that these drawbacks could be overcome if metaphase chromosomes are replaced by specific nucleic acids arrayed on a suitable medium as the hybridization target [106, 107]. The analysis of CGH experiments on such a format does not require identification of banded chromosomes, increases the resolution essentially to the length of the blotted nucleic acid sequences, allows automation, and with the genome sequence available enables investigators to seamlessly identify the nature of the genes affected by copy number imbalances. Initially the concept of array-based CGH was realized using BAC clones immobilized on glass slides. While suitable for detecting gene-specific copy number variations, improvements in this technology now utilize defined oligonucleotide sequences, either synthesized or printed onto slides, or attached to beads, to provide remarkably robust and reproducible tools for the high-resolution mapping of genomic imbalances in test genomes. Many of these arrays not only allow detection of copy number changes, but also identification of sequence polymorphisms, to provide information on allelic imbalances (loss of heterozygosity). The application of aCGH to cancer samples of the same entities as analyzed by CGH ) confirmed the predominance of recurrent, cancer-specific chromosomal gains and losses (Fig. 4.2a). However, aCGH is particularly useful for the high-resolution mapping of amplified regions, and for the detection of subtle deletions (Fig. 4.2b). Furthermore, aCGH shows that chromosomal breakpoints in carcinomas (here specifically colorectal carcinomas) map to the site of copy number variants (CNVs) in the human genome [108] (Fig. 4.2c). Only high-resolution platforms enabled the detection of such unrecognized plasticity of the human genome in cancer cells.


Fig. 4.2
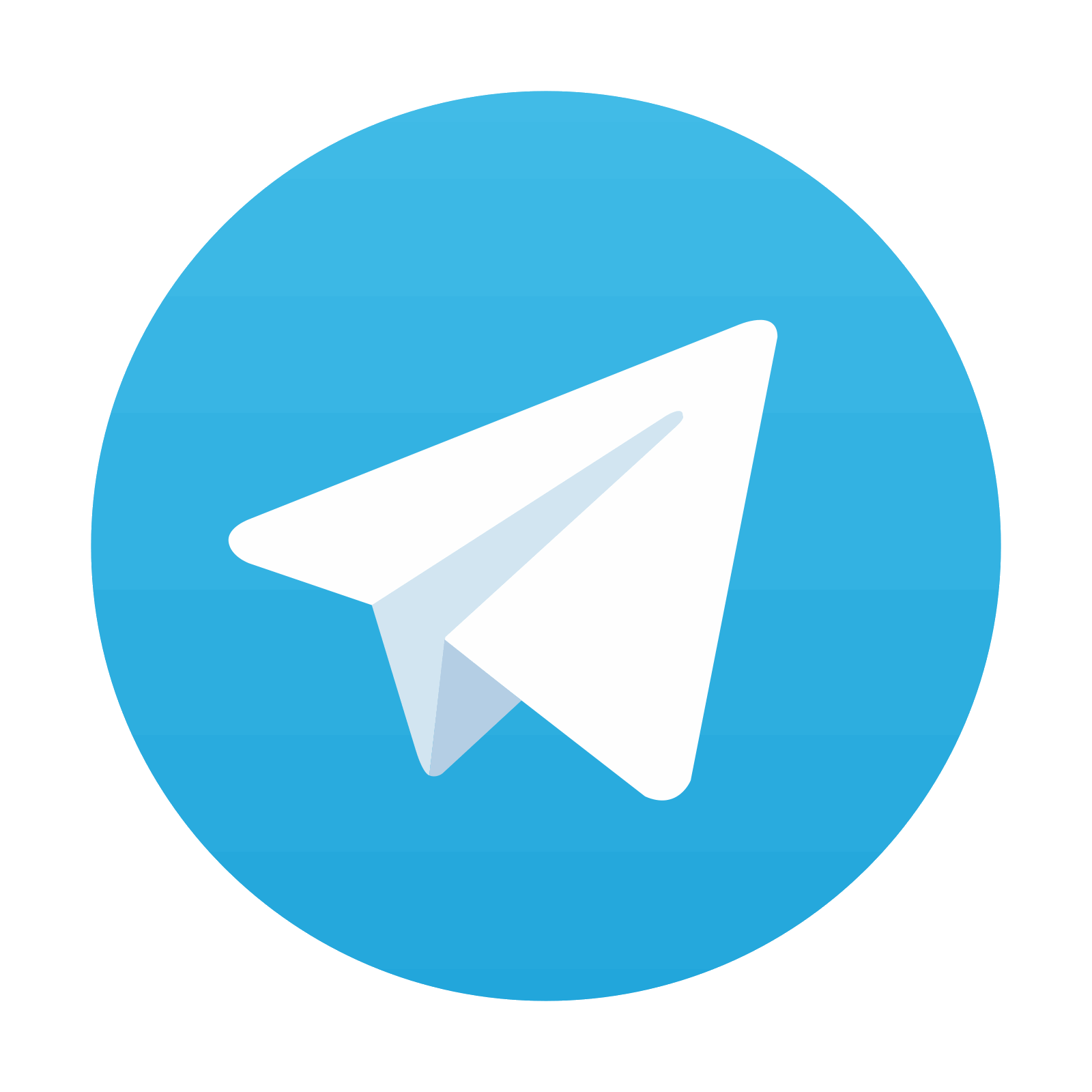
Methods and applications of molecular cytogenetics. (a) aCGH analysis of colorectal carcinomas. Shown is a map of chromosomal gains and losses over the entire genome. The vertical dotted lines demarcate individual chromosomes. The distribution of genomic imbalances is strictly conserved and specific for this cancer entity. For instance, gain of chromosome 13 occurs only in CRC, but not in other cancers of epithelial origin. CRC is defined by gains of chromosomes 7, 8q, 13, and 20q, and losses that map to chromosomes 8p, 14, 15, 17p, and 18q. This CRC-specific distribution of whole and partial chromosomal gains and losses is maintained in local and distant metastases, and in cell lines derived from primary cancers. (b) High-resolution mapping of an amplicon in CRC. In this case, the MYC oncogene is part of a localized high level amplification on 8q24, as indicated. aCGH also identified a small deletion (green dots) and a copy number gain (red dots) towards the telomere of the chromosome. (c) Structural variants in the human genome are prone to cause cancer initiating chromosomal aberrations . In this case, the deletion of the APC tumor suppressor gene, which is frequently inactivated in CRC, was likely triggered by recombination events at the site of a copy number variant (CNV). Possibly, replicative stress at these sites can induce double strand breaks which can result in chromosomal aberrations. (d) SKY analysis of the most malignant brain tumor, glioblastoma multiforme. The poor prognosis of this tumor is paralleled by the enormous amount of genomic instability, accentuated by the fact that most chromosomes are involved in rearrangements. Yet chromosome territories are clearly demarcated in the interphase nuclei (incomplete in the lower left and middle right section of the image). (e) SKY analysis of mouse chromosomes. The image shows normal murine chromosomes. SKY was particularly helpful in validating mouse models of human cancer, because it allowed a rapid assessment of chromosomal instability in cells prepared from a greatFig. 4.2 (continued) number of mouse models. Mouse chromosome analysis based on banding alone is challenging because all mouse chromosomes are acrocentric and less different in length compared to human chromosomes. (f) A tripolar mitosis in cancer cells (note the similarity with Fig. 4.1c). Alpha tubulin (stained in green) labels microtubules required for proper chromosome segregation upon attachment to the centromeres of metaphase chromosomes. DNA is stained in red. In this case the deletion of the tumor suppressor gene BRCA1 led to an amplification of centrosomes that caused abnormal mitoses. The DNA content of the daughter cells will not be normal (one of Boveri’s hypotheses). (g) Consequences of chromosomal aneuploidies on the transcriptome. This figure visualizes the result of a chromosomal trisomy on transcriptional activity of genes on that chromosome. The trisomy was induced using microcell-mediated chromosome transfer. Gene expression profiling demonstrated that the expression levels of most genes are dependent on genomic copy number (lower profile). This is consistent with observations in primary cancers or cell lines. (h) FISH on tissue sections. Shown is the amplification of the cell cycle regulating gene CCND1 in a tissue section of a head and neck cancer (this malignant neoplasm was located in the tongue) in highly aneuploid nuclei. Diploid copy numbers of this gene are present in lymphocytes in the lower left corner of the image. (i) Extra copy numbers of chromosome 3q are always present in cervical cancer. This figure shows cervical epithelial cells collected at a routinely performed Pap smear. The arrow denotes a normal diploid cervical cell, with two copies of the long arm of chromosome 3q probed using the human telomerase gene TERC (green) and a control probe for the centromere of chromosome 7 (red). The cells with an aberrant hybridization pattern all show six copies of TERC, which clearly suggests a clonal expansion once the cervical cancer specific aneuploidy was acquired. (j) Phenotype/genotype correlation of a high-grade cervical dysplasia (high-grade squamous intraepithelial lesion, or HSIL). This Pap smear was repeatedly assessed as being normal, yet was followed by the diagnosis of an invasive cervical carcinoma. Retrospective analysis of this case clearly demonstrates the potential of molecular cytogenetics in augmenting the morphology-based evaluation of cytological specimens. The presence of four copies of TERC is obvious. Again, the emergence of cervical cancer is consistent with a clonal expansion of cells that acquired the gain of 3q.
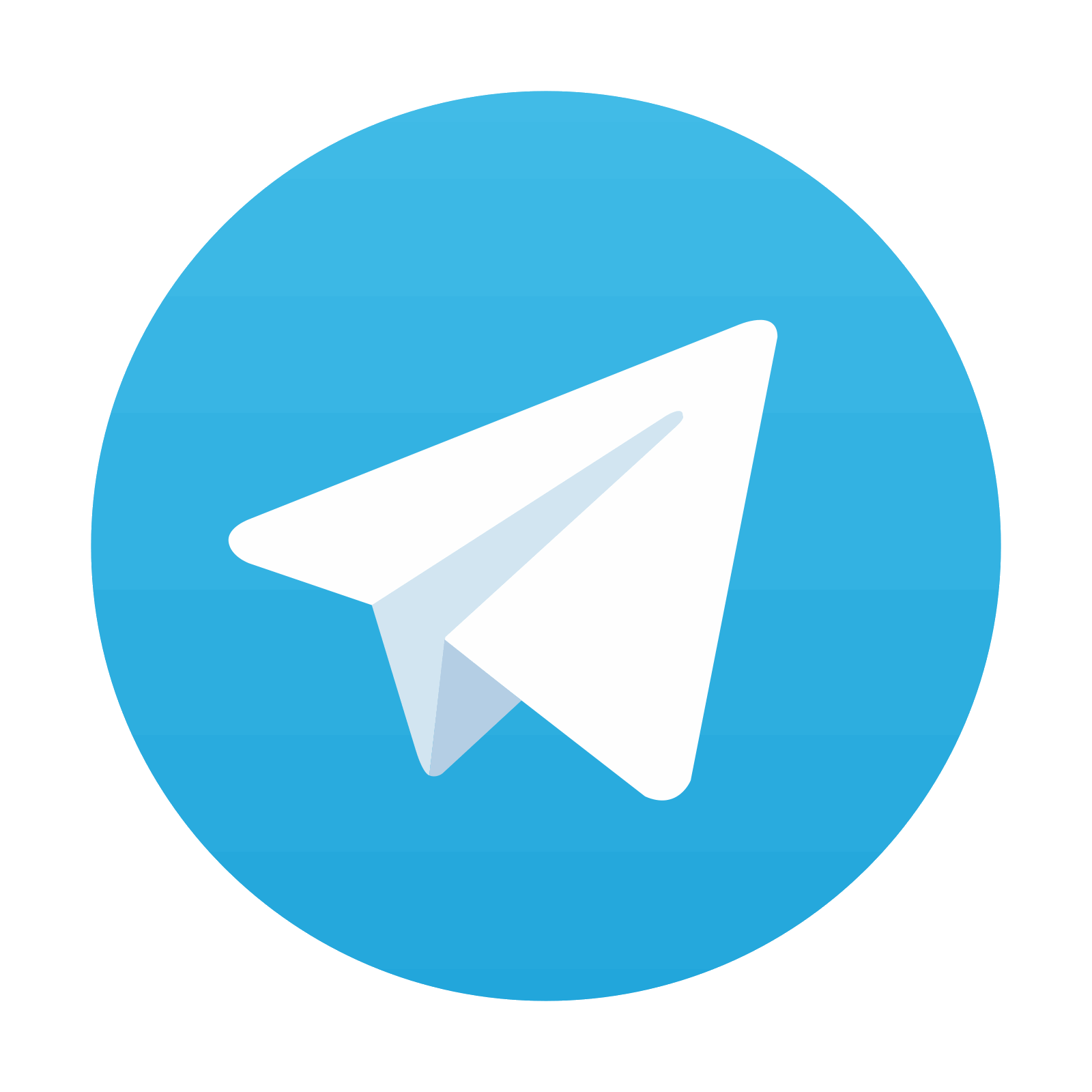
Stay updated, free articles. Join our Telegram channel
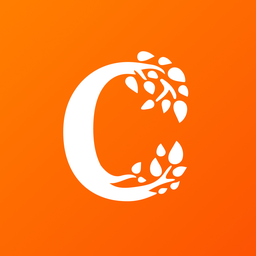
Full access? Get Clinical Tree
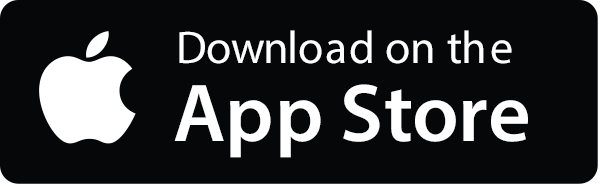
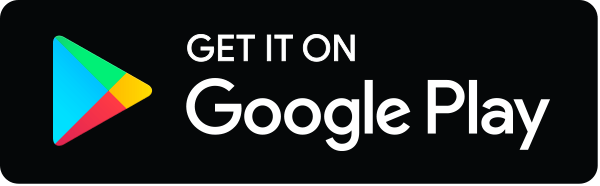
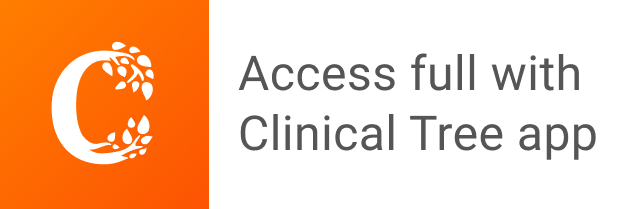