6.1 Introduction
B lymphocytes play a crucial part in adaptive immunity. They give rise to plasma cells that secrete antibodies, as well as memory B cells. These are crucial for immediate and long-term defence against many infectious agents. B cells appear to have co-evolved with T cells. Antibodies are structurally very similar to T cell receptors (TCRs) and the processes that generate the enormous diversity of antibody specificities in B cells are very similar to those that occur in T cells. We do not fully understand how two different types of lymphocyte appeared in evolution, but it is clear that B and T cells do recognize antigens in very different ways. This evolution may have been facilitated by two entire genome replications that occurred before and during the Cambrian explosion some half a billion years ago. The duplicated sets of chromosomes now enabled a group of genes on one chromosome to diversify from their original forms and to assume new functions. This may have allowed the generation of closely related but different types of antigen receptor during evolution of the two main types of lymphocyte.
In this chapter we discuss the functions of different types of antibodies in host defence, and describe how B cells can make different types of response against infectious agents. We start by noting that there are different types of response against B cells that can develop into plasma cells that make different types of antibodies, sometimes against different classes of molecular structures (Section 6.1). We then explain how it is possible for antibodies to recognize so many different kinds of molecule, before considering the different types of antibody, where they are commonly found and how they help in host defence against infection (Section 6.2). We then describe how different types of B cell can recognize different types of infectious agents and produce antibodies against them in different ways – and how in many cases they need to collaborate with T cells in order to synthesize the most appropriate type of antibody. We describe the anatomy and physiology of B cell activation and antibody synthesis (Section 6.3), and show how long-term resistance to infection can occur through antibodies (Section 6.4). We explain how and where different types of B cell develop and why it is that antibodies do not normally recognize components of the host itself, a part of immunological tolerance (Section 6.5). Finally, we outline how antibodies can be produced and used as tools for the treatment of diseases (Section 6.6)
6.1.1 B Cell Populations
B lymphocytes are the precursors of antibody-forming cells, known as plasma cells, and of memory B cells. In contrast to T cells that can develop into subsets (Th1, Th2, etc.) with very different functions, the major variation in the effector function of B cells lies in the biological properties of the different classes of antibody that they can secrete. In humans and mice, for example, these are the IgM, IgD, IgG, IgA and IgE isotypes. However, different subsets of CD4 T cells often control which class of antibody a plasma cell will secrete. B cells also differ from T cells in that they can change the structure of their antigen receptors, and thus of the antibodies they secrete, by somatic mutation after they have been appropriately stimulated by antigen.
Morphologically, newly produced, naïve B cells are indistinguishable from T cells. However, they do of course have a very different pattern of gene expression, and because they express different surface molecules they can be readily distinguished from T cells and other mononuclear cells. In mice and humans there appear to be three main populations of mature B cells:
Follicular B cells and marginal zone B cells are sometimes referred to as B-2 cells to distinguish them from B-1 cells (above). Because they need help from CD4 T cells, follicular B cells typically take longer to make antibody responses than the other types of B cells. In contrast, marginal zone B cells can rapidly make antibody responses to TI antigens, while some B-1 cells may continuously and spontaneously produce natural antibodies. See Figure 6.1.
Fig. 6.1 B cell populations. The same B cell precursor can probably give rise to different B cell populations. All subsets initially express IgM and most express IgD. Activated follicular B cells can develop into plasma cells that secrete IgM and some IgD (not shown). These B cells can also switch the class of immunoglobulin they express to IgG, IgA or IgE and can develop into plasma cells or memory cells. Activated marginal zone B cells can become plasma cells that make IgM antibodies, typically to carbohydrate antigens, but they do not switch to most other immunoglobulin classes or become memory cells. Some B-1 B cells make natural IgM antibodies in the apparent absence of any antigenic stimulation and perhaps some IgA.
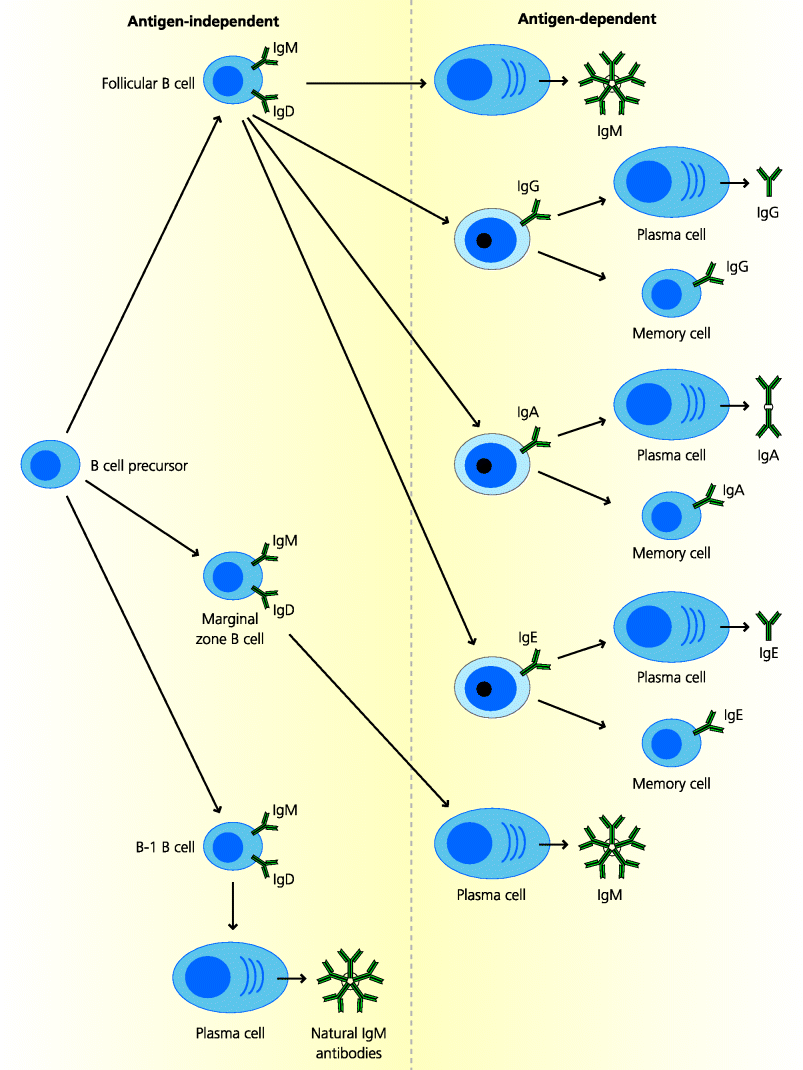
6.1.2 How Do Antibodies Recognize Antigens?
B cells recognize antigens through their membrane-bound B cell receptors (BCRs). These receptors are secreted in a soluble form, as antibodies, after the B cells develop into plasma cells. What is the range of antigens against which B cells can make antibodies? Collectively, B cells can respond to a huge range of molecules including proteins, carbohydrates, nucleic acids, glycolipids or even small inorganic molecules such as dinitrophenol (DNP) which do not exist in nature. In contrast to conventional T cells which recognize linear peptides that can arise from any part of a protein, (sequential epitopes), bound of course to MHC molecules antibody molecules recognize small parts that are on exposed surfaces of a molecule called conformational epitopes. These epitopes need to possess a stable three-dimensional structure if antibodies are to be made against them. X-ray crystallography has enabled the different types of atomic interactions that can occur between antibodies and their corresponding antigens to be visualized, and has shown the variety of shapes that can be adopted by the antigen-binding sites of antibody molecules.
6.2 Antibody Structure and Function
6.2.1 Immunoglobulins and Antibodies
The terms immunoglobulin and antibody are often used interchangeably. Strictly, however, immunoglobulin is the name given to a particular group of protein molecules in blood plasma or secretions with a size and electrophoretic mobility that characterise them as the γ-globulins. Antibodies are immunoglobulins for which an antigen has been identified (i.e. antibody is really a functional label). The term antigen was initially used to describe anything that triggered the production of antibodies. Now, however, this term is more generally used to describe anything that can be recognized by a lymphocyte, including T cells.
Immunoglobulins are made of different polypeptide chains: the heavy (H) and light (L) chains. Each immunoglobulins chains has a characteristic structure: they are built from a number of domains, which possess common features in their three-dimensional conformation. This general structure is known as the immunoglobulin domain. It is characterized by the immunoglobulin fold, a particular conformation of closely apposed β-pleated sheets that forms the structure of each domain. The immunoglobulin domain is not only found in antibodies. Many other molecules, often involved in recognition, including TCRs, CD4 and CD8, and the co stimulatory molecules involved in T cell responses (Chapter 5), are made up of immunoglobulin domains. Thus we can deduce that the immunoglobulin domain is evolutionarily ancient. The Thy-1 protein (CD90) is one of the most ancient; it contains a single immunoglobulin domain and is present in all vertebrates and even in squid, an invertebrate. All molecules containing immunoglobulin domains are members of the immunoglobulin superfamily. As happens so often the adaptive immune system has utilised and diversified a pre-existing structure for its own purposes.
6.2.2 Antibody Structures
The characteristic structure of antibodies was initially worked out using biochemical techniques. We now know that the classical Y-shaped antibody monomer is composed of two H chains and two smaller L chains that are disulphide linked to each other (Section 1.5.3.2). Each H and L chain has one portion, called the variable (V) region, that come together to form the binding site for antigen, so that each immunoglobulin monomer is bivalent. Each V region contains three stretches of amino acids that differ greatly in sequence between antibodies of different specificities, and are therefore known as hypervariable regions. In an antibody, when a H chain V region combines with a L chain V region, these hypervariable regions are brought together to form the antigen-binding site and thus determine the antigenic specificity. As the binding of antibody to antigen is controlled by complementarity in shape, the hypervariable regions are also called complementarity-determining regions (CDRs). They are numbered CDR1, CDR2 and CDR3 for each chain. Similar principles apply to TCRs Section 1.5.3.2. See Figure 6.2.
Fig. 6.2 Antigen-binding sites. When an immunoglobulin molecule folds into its tertiary structure, the six hypervariable regions (CDRs) – three each from the H and L chains – are brought together, they form the three-dimensional antigen-binding site, which can vary in structure from a flat surface as shown or a deep cleft (not shown).
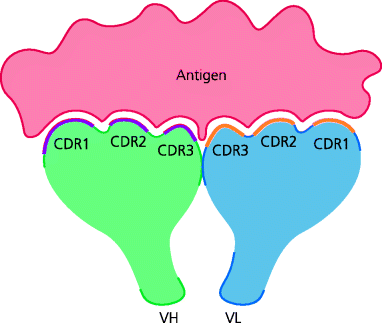
The remaining portions of the H and L chains are called constant (C) regions. For H chains in humans and mice, there are five main C regions, called µ, δ, γ, α and ε. These define the class (isotype) of antibodies produced – IgM, IgD, IgG, IgA and IgE, respectively. There can two or more forms of different classes of antibodies, particularly IgG and sometimes IgA, and these are called subclasses. For L chains there are two main types of C region, κ and λ, and the corresponding chains that contain them are named accordingly. The C regions of the H chain are entirely responsible for the different tissue localizations and effector functions of the different antibody classes; whether a κ or a λ C region is present in the L chain of an antibody does not seem to have any biological effect at all See Box 6.1.
6.2.3 Generation of Antibody Diversity
It was apparent from early days that any individual could make antibodies against any of a huge number of different antigens. Since these antibodies did not generally cross-react with other antigens it was argued there must be at least an equally large number of antibody genes. At the time it was very difficult to understand the genetic basis of this antibody diversity. Estimates suggested that humans or mice could make more than 107 antibodies. Did this require 107 genes? This was far beyond the number of genes that had been estimated to exist in the genome. In fact, we know this to be impossible because the human genome project has revealed that humans have only around 30 000 genes in total. Many theories were put forward to explain this paradox, and it was not until the mid-1970s that the answer was found.
Using molecular biological techniques (Southern blotting) it was shown that immunoglobulin genes were arranged differently in B cells compared to other cells types. It was found that segments of antibody genes that were widely separated in the germline DNA of other cells were closely linked in B cells. This suggested a mechanism in which the complete immunoglobulin gene present in a B cell was constructed by bringing together different segments of germline DNA. If there were multiple variants of each segment, a huge number of different antibody genes could be made by random combinatorial association of a relatively small number of segments. Further analysis by many groups showed that this hypothesis was correct.
It is now clear that complete immunoglobulin genes are constructed from different gene segments in humans and mice by rearrangement of DNA. The mechanism by which immunoglobulin gene (and TCR gene) rearrangement occurs in species such as humans and mice is termed somatic recombination because it occurs involves recombination of stretches of DNA in somatic cells rather than germ cells. Generally, this form of DNA rearrangement occurs during the early differentiation of B cells (and T cells) into mature lymphocytes (Section 1.5.3.2).
6.2.3.1 Rearrangement of Immunoglobulin Genes DNA
DNA coding for immunoglobulin genes exists as discrete groups of V, D and/or J, and C gene segments in the genome. A complete H chain gene is made by bringing together one segment from each of the V, D and J groups. The selection of an individual segment from a group is largely at random. The V region segments initially become associated with two of the H chain C region gene segments (µ and δ). Following B cell activation the cell may switch by associating a different C region (γ, α or ε) with its V region gene. The L chain V region genes are similar, but are made from only two segments, V and J. The V region gene segments will associate with either a κ or λ C region segment; κ and λ gene segments are present on different chromosomes. The number of gene segments in each group differs. Thus, in the human H chain region, there are around 40 V segments, 27 D segments and six J segments. In the human L chain regions there are around 40 (κ) or 30 (λ) V segments and five or four J segments. See Figure 6.4.
Fig. 6.3 Studies of immunoglobulin structure. When antibodies were digested with the protease papain, fragments of two sizes were obtained. One fragment could bind antigen and was called Fab; this fragment could not be crystallized. The other fragment did not bind antigen but could be crystallized and was called Fc. If however, immunoglobulin was digested with pepsin, a single large fragment was obtained that could bind two antigen molecules and was called F(ab′)2. When disulphide bonds were reduced, two complete H and L chains were released from each antibody monomer. The V and C regions of each chain are indicated.
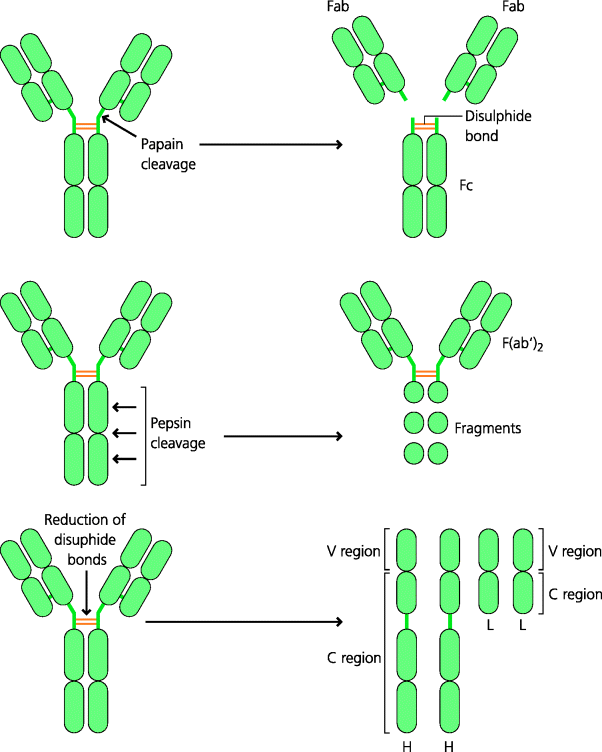
Fig. 6.4 Generation of immunoglobulin genes. Complete immunoglobulin genes are found only in B cells. In germline DNA, three separate loci on different chromosomes code for the H chain and the κ and λ L chains. At each locus there are multiple gene segments that code for parts of the V region (and C regions). The H chain region contains numerous VH segments, a number of DH segments and a small number of JH segments. In B cells, one segment from each group is selected (largely randomly) and the selected segments are brought together to form the complete V region. The V region is paired initially with the µ (and δ; not shown) C region segments. For L chains the principle is similar, but there are no D region segments and the V region pairs with either κ or λ C region segments. During assembly of the complete H and L chain genes, additional bases can be inserted at the junctions between V, (D) and J segments, increasing junctional diversity (see Chapter 5, Figure 5.28 for details). The order of the C region genes is shown in a simplified form for humans.
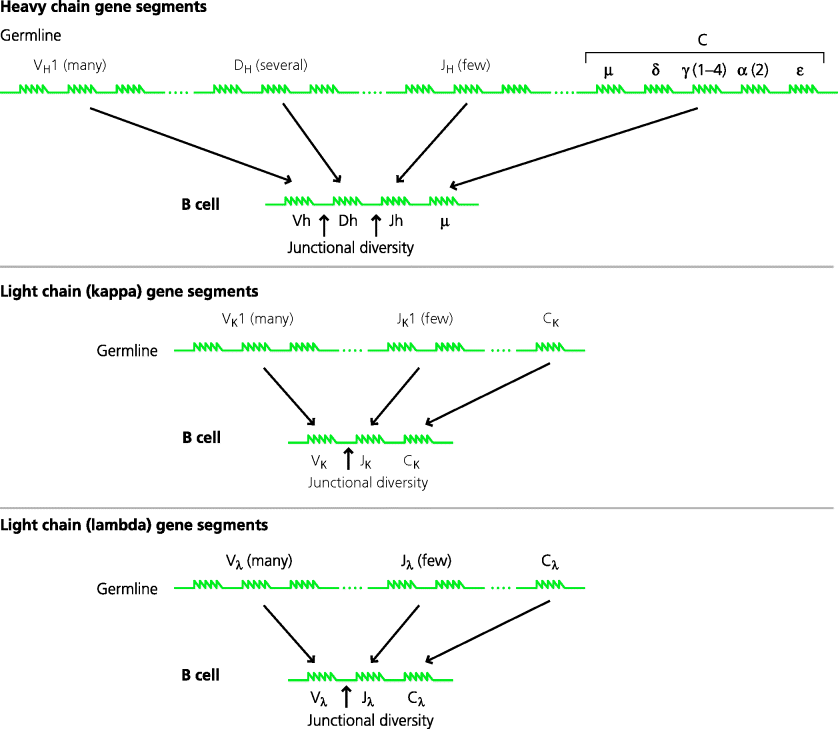
The order in which the different immunoglobulin gene segments rearranged could be established by examining populations of B cells at different stages of development. In some cases, examination of B cell tumours, in which the cells are arrested at a particular stage in development, was also very informative. We now know that rearrangement of the H chain segments occurs first and this is followed by the L chain. For H chains, one D segment joins to one J segment and this rearranged DJ segment then joins to one V segment. In L chains, there is just V–J joining. For both H and L chains, the respective V gene segments encode both CDR1 and CDR2 while the junctions (from V to J, with or without D depending on the chain) encode CDR3. If all the V, D and J segments were used, they could generate around 6500 H chains and 200 L chains. Since any recombined H chain can in theory join with any L chain, this combinatorial process could generate around 1.3 × 106 antibody specificities. However, the actual potential diversity is much, much higher because additional mechanisms can introduce further variability (below).
Two important enzymes involved in joining the different V gene segments are the recombinase-activating gene (RAG) endonucleases RAG-1 and RAG-2. These proteins recognize recombination signal sequences (RSSs) (Section 5.5.1.3) that flank the gene segments, and these sequences are arranged so that the immunoglobulin gene segments can only join appropriately. So, for example, a H chain V segment is normally prevented from joining directly to a H chain J segment. Human patients who possess a defective RAG gene are unable to make either BCRs or TCRs, and the developing lymphocytes stop further differentiation and die. As these patients lack mature lymphocytes, they are highly susceptible to life-threatening infections; they suffer from severe combined immunodeficiency(SCID). That a RAG gene defect is sufficient to cause SCID has been confirmed in mice in which one of the RAG genes has been knocked out these mice have neither T nor B cells.
As mentioned, several other mechanisms contribute to diversity by varying the nucleotides present at the junctions between the gene segments. This junctional diversity is introduced by two different processes (Figure 5.28). First, an enzyme called Artemis acts after RAG during the joining process and may generate staggered breaks in the DNA. Depending on how this happens subsequent DNA replication at these sites can generate palindromic sequences, which are called P-regions. Not surprisingly, gene defects in Artemis are another cause of SCID. Second, another enzyme, terminal deoxynucleotidyl transferase (TdT), can insert additional nucleotides into the junctional sites and this gives rise to so-called N-regions. The generation of P- and N-regions vastly increases the diversity that can be created during the construction of immunoglobulin genes and hence of the antibodies that can be formed. In humans, it is estimated that around 5 × 1013 different antibodies could potentially be generated; in contrast, the total number of B cells in an adult human is probably less than this by at least two orders of magnitude.
6.2.3.2 Allelic and Isotype Exclusion
All vertebrate somatic cells (i.e. other than the sperm and egg germ cells) are diploid; in other words, they possess a set of chromosomes from both parents. In theory it would be possible for the maternal and paternal immunoglobulin V gene segments of both the H and L chains to be rearranged and expressed in any given B cell, and to make and express several antibodies of different specificities. We know, however, that each B cell only makes one antibody specificity. The reason for this is, in part, that if there is a successful rearrangement on one chromosome, this inhibits rearrangement on the other chromosome, so either the maternal or the paternal allele can be expressed, but not both. This process is called allelic exclusion. In mammals, allelic exclusion only occurs for these immunoglobulin genes, the TCR β (but not α) genes, and the nasal olfactory receptors for the sense of smell. Moreover, because there are two different L chains loci (κ and λ) it is essential that if one rearranges successfully, the other is inhibited. This is called isotype exclusion. (It is of course also essential that rearrangement stops as soon as a functional immunoglobulin chain is produced from any locus, otherwise a B cell could keep producing an almost limitless number of antibody specificities during its life.)
6.2.4 Antibody Classes (Isotypes) and Their Properties
As we outlined above, antibodies can be grouped into five main immunoglobulin classes or isotypes by the characteristics of the C regions of their H chains. In some cases there are different versions of some of the isotypes called immunoglobulin subclasses. It is the C region that determines all the biological properties of an antibody, other than its antigen-binding specificity, and it is these properties that determine how and where antibodies work in defence against infection. See Figure 6.5.
Fig. 6.5 Structures of different classes of antibodies. The five main classes of antibodies all have a similar monomeric structure. Some classes can also form multimers, such as IgM pentamers or IgA dimers in which the monomers are linked by another component called the J chain. However, the detailed structure of the monomers differs for each class (and subclass) of antibody. These differences lead to different properties for each antibody. For example, the arms of the monomer may be relatively rigid or flexible. The stem may be short or extended. The biological properties of antibodies are determined by the structure of the stem, enabling it to be recognized by different types of FcR, for example, or to activate complement. (Immunoglobulin subclasses for IgG and IgA are not shown.)
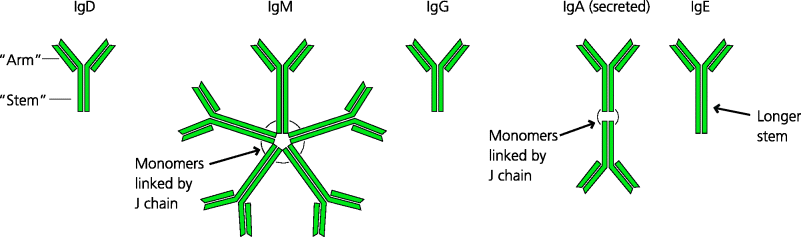
6.2.4.1 IgM
All naïve B cells express surface IgM (they also co-express IgD of the same specificity; below). Secreted IgM is composed of five immunoglobulin units that form a pentamer joined by a polypeptide chain, the J chain. Thus, IgM is a very large molecule with 10 potential antigen-binding sites (in most cases an IgM molecule cannot bind 10 antigen molecules because of steric interference). Each binding site will have an identical affinity for an antigen, but multi-valency dramatically increases the avidity of the IgM molecule for its antigen.
Fig. 6.6 Antibody affinity and avidity. The affinity of an antibody refers to the strength of binding of a single antigen-binding site to a single antigenic determinant (epitope). Antibody avidity refers to the overall strength of binding between all the antigen-binding sites and an antigen that expresses more than one identical epitope. The avidity of a divalent F(ab′)2 fragment is vastly greater than twice the affinity of a monomeric Fab fragment of the same antibody. Likewise, the avidity for a divalent IgG antibody may be 100 times its affinity, and for pentameric IgM 1000 times or more.
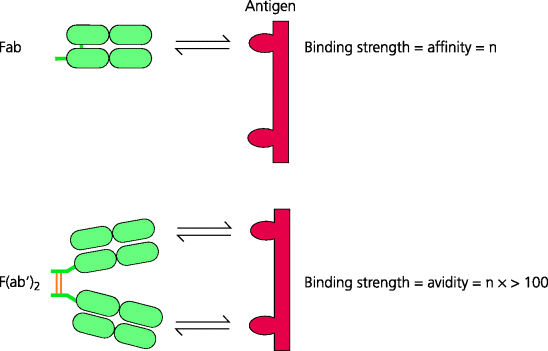
Under normal conditions, IgM is found only in the blood because it cannot cross the endothelial barrier. You will, however, remember that during acute inflammation, venule endothelial cells separate from each other and this allows IgM to enter extravascular inflamed tissues. How does IgM act in defence? Its main role is against pyogenic infections and, as we describe in Section 2.3.1.3, the main type of effector cell for these infections is the neutrophil. Phagocytes, such as human neutrophils, do not have Fc receptors (FcRs) for IgM, and IgM thus cannot act as a direct opsonin. IgM is, however, a very efficient complement activator. As secreted IgM is pentameric it has five Fc regions in very close proximity to each other, and this allows the C1q complement component to bind and to trigger the cascade (Section 4.4.2.1). Neutrophils have receptors for derivatives of the C3 complement component (C3b and iC3b), and thus bacteria coated with IgM and complement can be phagocytosed via complement receptors (Figure 2.10).
Natural Antibodies
Natural antibodies are so-called because they are formed without any obvious or overt stimulation by foreign antigens. They are mainly IgM; however, some IgG, particularly IgG3 in mice and humans, and perhaps some IgA may also fall into this category. Natural antibodies are broadly reactive (i.e. they react with a range of pathogens with relatively low affinity). They may also be involved in autoimmune diseases such as systemic lupus erythematosus (SLE, Section 7.4.3). Natural antibodies do appear to have a role as first-line defence against infection. For example, they may be able to neutralize bacteria and viruses present in blood. Natural IgG antibodies may also be involved in the induction of some types of TI responses, by binding to blood-borne antigens and targeting them to cells, possibly macrophages, in the marginal zone in the spleen. Some natural antibodies may be autoreactive and it has been suggested that these may be involved in clearance of debris from self cells, and even that failure of this clearance may lead to autoimmunity but this is controversial.
Natural antibodies are formed primarily by a subset of CD5 B-1 cells (called B-1a cells) in the peritoneal and pleural cavities, at least in mice. They are encoded by immunoglobulin genes that preferentially use certain V regions and, because little or no TdT is expressed by these cells, they have very limited N-region junctional variation; they also do not undergo somatic hypermutation (Section 6.3.3.8). Their formation is independent of overt antigenic stimulation and in germ-free mice they are present at similar concentrations to normal mice. Natural antibodies thus in some ways represent an innate immune mechanism, although their efficacy depends on their binding to antigens through a variable antigen-binding site, normally thought of as a characteristic of adaptive immune responses.
6.2.4.2 IgD
For many years IgD has been a mystery molecule. It differs from all other immunoglobulin isotypes in that only trace amounts are normally found in the blood (some rare myelomas do, however, secrete large quantities of IgD). IgD is expressed on the surface of most naïve follicular B cells, together with IgM. This is because a rearranged V gene approximated to the µ and δ constant genes comprises a single transcriptional unit, and one or the other can be produced simply by alternative splicing of the mRNA that encodes them. Genetically engineered mice that lack IgD have lower than normal levels of B cells, affinity maturation (Section 6.3.3.8) of their antibody responses is delayed and they also produce lower levels of immunoglobulin isotypes such as IgE that are controlled by the cytokine IL-4 (Section 6.3.3.6). Recently it has been shown that secreted IgD can be produced by class switching, involving DNA rearrangement, that human basophils possess a receptor for IgD and that binding to this receptor can trigger basophil responses, at least in the upper respiratory tract. It therefore seems that IgD may play a role in defence against respiratory bacteria, and may have other functions in immunity and inflammation that are yet to be discovered.
6.2.4.3 IgG
IgG is the most abundant class of antibody in the blood. IgG is a monomeric molecule that comprises several different subclasses with different Fc regions (four in humans and mice). These subclasses have some differences in function such as the ability to activate complement and trigger inflammation, or to directly opsonize microbes for FcR binding (Figure, 1.31 and 2.10). Unlike IgM, most IgG synthesis is CD4 T cell-dependent. Which subclass is produced during adaptive immune responses depends largely on the type of CD4 T cell response that is involved (e.g. Th1 versus Th2; see later).
IgG is crucially important for defence against infection in early life, before the adaptive immune system is fully developed. In mice, rats and humans, IgG can cross the placenta via a specialized transport molecule, the neonatal FcR, making it available for protection of the late foetus. Because maternal IgG lasts for several months in the offspring’s circulation and tissues it also provides defence for the new-born infant (as does IgA from the colostrum, early milk; below). It is for this reason that the severe infections that are seen in human primary immunodeficiencies such as SCID (above) often do not become apparent until several months after birth, when the maternal antibody has disappeared. See Figure 6.7.
Fig. 6.7 Tissue distribution of antibodies. IgM is confined to the blood plasma unless there is acute inflammation, when it can pass between endothelial cells into extravascular spaces. IgG can cross endothelia into many extravascular spaces and, in addition, can cross the human placenta to give protection to the neonate. Monomeric IgA tends to be present in the blood, but dimeric IgA is transported onto mucosal surfaces such as the gastro-intestinal, respiratory and uro genital tracts, and the lactating breast. IgE is normally present at very low concentrations in blood but binds to mast cells, particularly those underlying the epithelia. IgD is usually only present in significant amounts on the surface of naïve B cells and is normally only present in the blood at exceedingly low levels; not shown.
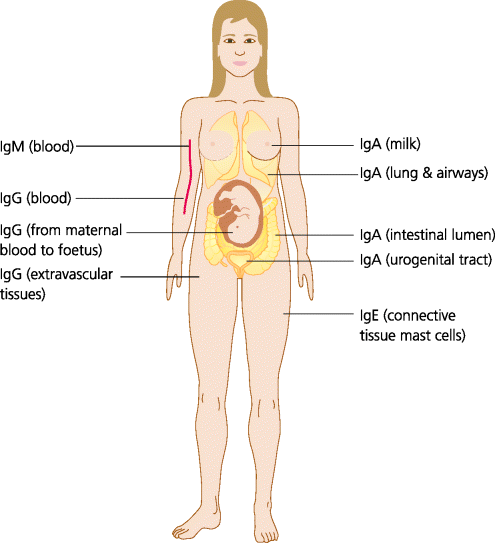
6.2.4.4 IgA
IgA is the most important mucosal antibody. Most IgA is secreted by plasma cells in the connective tissues underlying mucosal epithelium in tissues such as the intestine, the respiratory tract, the uro-genital tract and the lactating mammary gland. IgA is secreted into the connective tissue, but is then actively transported (below) across the epithelial cells into the lumen overlying the epithelium, which is where it carries out its main protective functions. The most important action of IgA is to prevent pathogens and toxins binding to epithelial cells (Figure 2.24). Much IgA is secreted in these sites as a dimer, with the two monomers joined by a J chain (the same molecule that joins IgM monomers to form a pentamer). IgA in blood is mostly found as a monomer. The functions of this form are not entirely clear although it can activate the alternative pathway of complement, and some macrophages possess FcRs for IgA, suggesting it may be involved in opsonization of infectious agents. Two subclasses of IgA exist in humans, both being present in the blood and secretions. One subclass (IgA2) lacks a stretch of 13 amino acids present in the other and shows increased resistance to some bacterial proteases; this may increase its potency on mucosal surfaces. IgA deficiency is one of the commonest immunodeficiencies. In the developed world around 1: 500 people are found to be IgA-deficient. Most are asymptomatic, but some suffer from an increased incidence of respiratory and intestinal infections.
Mucosal Immunity
Most infections enter the body through mucosal surfaces. It is thus not surprising that the adaptive immune system has evolved specializations to protect these surfaces. Immune responses to mucosal pathogens may be initiated in draining lymph nodes as for other tissues, but mucosal surfaces such as the intestine possess specialized secondary lymphoid organs in their walls (Section 3.4.1). In the small intestine these are the Peyer’s patches Section 3.4.2.7.
The main function of the Peyer’s patches and similar lymphoid aggregates appears to be the indeuctioin of IgA synthesis. Antigens from the gut lumen enter Peyer’s patches via M cells and encounter dendritic cells (DCs) in the subepithelial areas (Figure 3.25). These DCs interact with recirculating naïve T cells and, unless there is very strong stimulation, induce Th2 differentiation. This may be because the DCs are conditioned by their environment to secrete IL-10 and transforming growth factor (TGF)-β. The activated T cells interact with naïve recirculating B cells, activate them and induce isotype switching to IgA. This switch depends on the T cells secreting cytokines such as IL-4, IL-5 (in mice) and IL-10. The activated IgA lymphoblasts do not secrete IgA locally; rather they exit the lymphoid tissue in the lymph and subsequently enter the blood. These lymphoblasts express adhesion molecules (such as the α4β7 integrin) that are complementary to vascular addressins expressed on mucosal venule endothelium. The most important of these addressins is the mucosal addressin cell adhesion molecule (MadCAM). The lymphoblasts can therefore migrate into mucosal connective tissues and mature into plasma cells secreting dimeric IgA.
Mucosal epithelial cells express a specialized receptor on their baso-lateral surfaces for dimeric IgA, as well as pentameric IgM, called the poly-immunoglobulin receptor. Binding of these antibodies to this receptor is dependent on the J chain present in multimeric IgA and IgM. Having bound the polymeric immunoglobulin, the immunoglobulin–receptor complex is endocytosed by the epithelial cells. Normally, endocytosed molecules are delivered to lysosomes and degraded. However, the vacuole containing the poly-immunoglobulin–receptor complex is treated differently. It is transported across the epithelial cell to the apical surface and its contents are released onto the mucosal surface and thus into the lumen. During transport the poly-immunoglobulin–receptor complex is cleaved, forming a complex which consists of the IgA plus part of the receptor; the latter cleaved fragment is known as the secretory piece. It is this complex that is released onto the mucosal surface, and the secretory piece additionally confers some resistance to proteolytic breakdown. See Figure 6.8.
Fig. 6.8 Transport of IgA onto mucosal surfaces. Mucosal plasma cells secrete IgA as dimers, joined by the J chain. Dimeric IgA binds to poly-immunoglobulin receptors on the baso-lateral surfaces of mucosal epithelial cells and is endocytosed. These vacuoles are transported to the luminal surface of the epithelial cell where the poly-immunoglobulin receptor is cleaved. This releases the dimeric IgA, with a portion of the receptor known as the secretory piece, which renders the IgA less susceptible to proteolytic degradation.
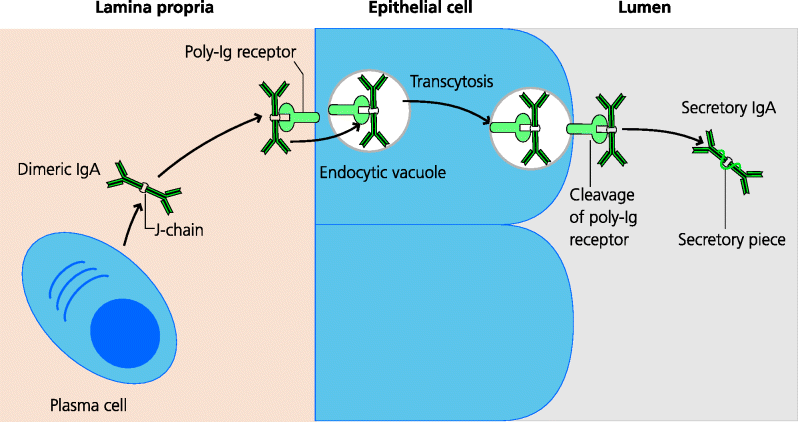
The Common Mucosal Immune System
The common mucosal immune system evolved so that immune responses initiated in one mucosal tissue can generate effector mechanisms, IgA in particular, that can operate in all mucosal sites. Some of the most dramatic examples of this come from studies on the protective effects of breast milk against intestinal infections. One study examined the incidence of diarrhoeal disease in young children in a large apartment block in Pakistan. The two groups of children being compared were those that were breast-fed and those that were not. The incidence of diarrhoeal disease was much lower in breast-fed children. This clinical observation is consistent with the concept of the common mucosal immune system: that immune responses induced in one mucosal site can lead to IgA (and activated T cells) being distributed to all mucosal sites. Thus, IgA-expressing B cells activated in the intestine against bacteria causing diarrhoea can migrate to the lactating breast, leading to IgA secretion into milk. These observations provide sufficient evidence to justify the encouragement of breast feeding in humans, particularly in the developing world. The common mucosal system can thus provide protection at all mucosal surfaces against an infection in just one site. See Figure 6.9.
Fig. 6.9 The common mucosal immune system. Antigens from the gut lumen, for example, cross M cells into Peyer’s patches (or similar secondary lymphoid organ in other mucosal sites) and, with T cell help, develop into B cells that express IgA. These cells then leave these secondary lymphoid tissues and enter the blood. They express homing receptors for adhesion molecules present on all mucosal venule endothelial cells and thus can migrate, relatively randomly, to any mucosal tissue. Within these tissues they mature into plasma cells secreting dimeric IgA which is transported onto the luminal surface of any mucosal tissue.
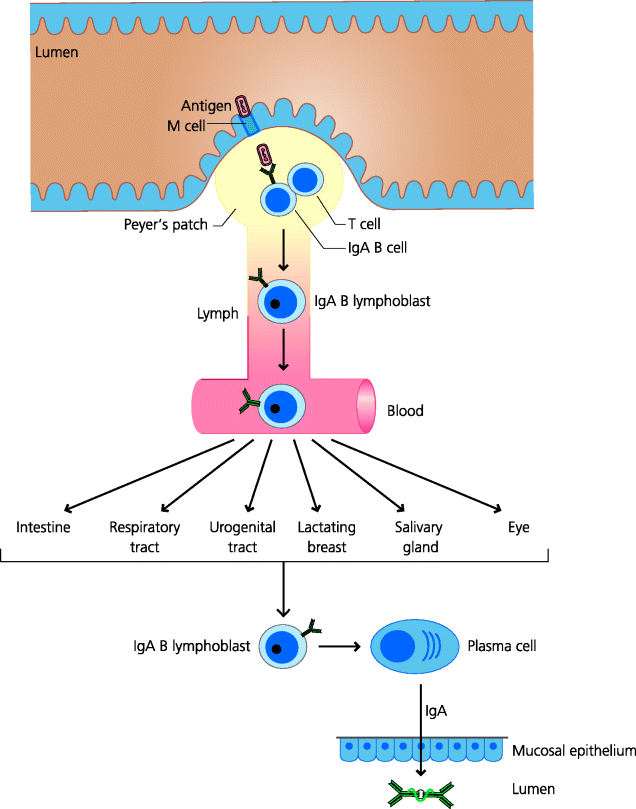
6.2.4.5 IgE
IgE is a monomeric molecule, but binds strongly to mast cells and eosinophils because these cells express a high-affinity FcR for IgE. IgE production and eosinophil recruitment are often associated. In Th2-biased responses B cells may switch to make IgE while eosinophils are being recruited to tissues in Th2-mediated inflammatory responses. IgE probably has a role in immunity to parasites and may act via eosinophils by activating them and stimulating them to degranulate and to kill parasites by antibody-dependent cell-mediated cytotoxicity (ADCC; below), but the relative important of this in host defence is not clear. All sufferers from hay fever should have an interest in IgE because it is the antibody responsible for allergic responses. These include potentially fatal conditions such as the anaphylaxis caused by peanut allergy (Section 7.2.3).
6.2.5 Monoclonal Antibodies
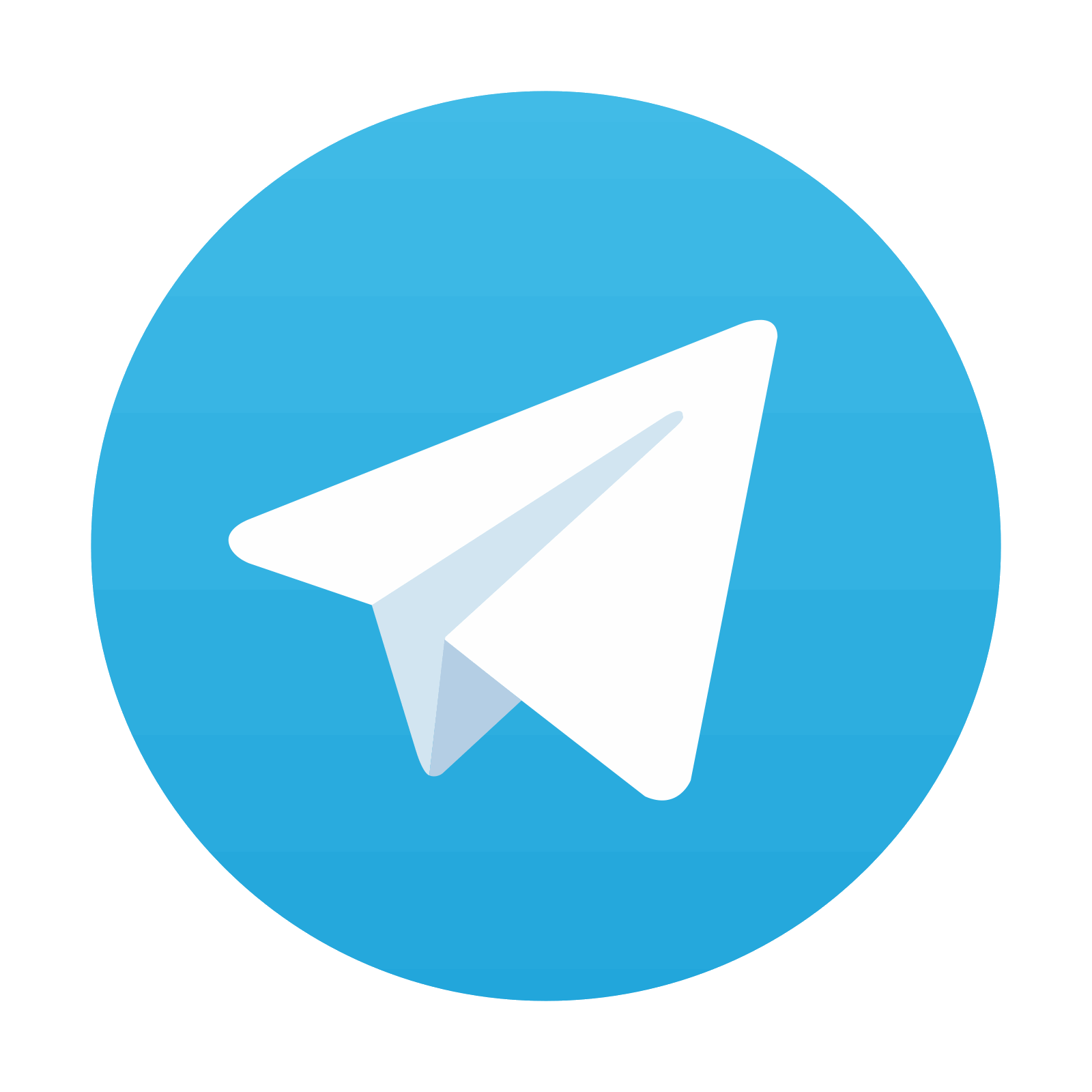
Stay updated, free articles. Join our Telegram channel
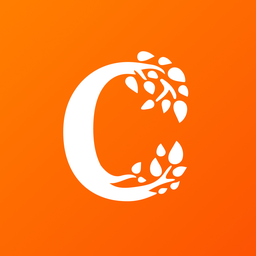
Full access? Get Clinical Tree
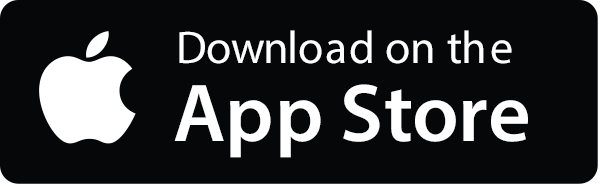
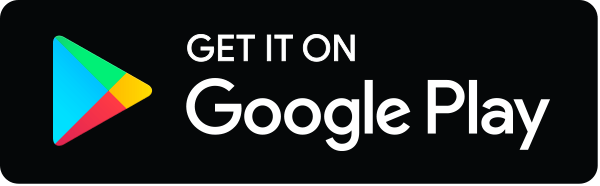