Fig. 16.1
A 21-year-old female with history of grade 3 pineal parenchymal tumor of intermediate differentiation (PPTID) status post gross total resection (GTR) receiving adjuvant whole ventricular radiation therapy. Top row: MRI images (axial, sagittal, coronal) corresponding to the planning CT scan (bottom row). The whole ventricular volume is outlined in yellow and the prescribed radiation dose in orange (Courtesy of Dr. Steve Braunstein, Department of Radiation Oncology, UCSF)
In the use of any of these conformal therapies, it is important to consider the relevant anatomical features. Particularly in the use of inverse planning, if an area is not contoured as tumor, it will likely receive suboptimal dose. Areas of subclinical disease must also be taken into account. Only through careful consideration of anatomy and dose can IMRT be safely applied. Once can achieve sparing of inner structures with IMRT, as compared to 3DCRT, without compromise of the dose delivered to the target volume (Fig. 16.2).
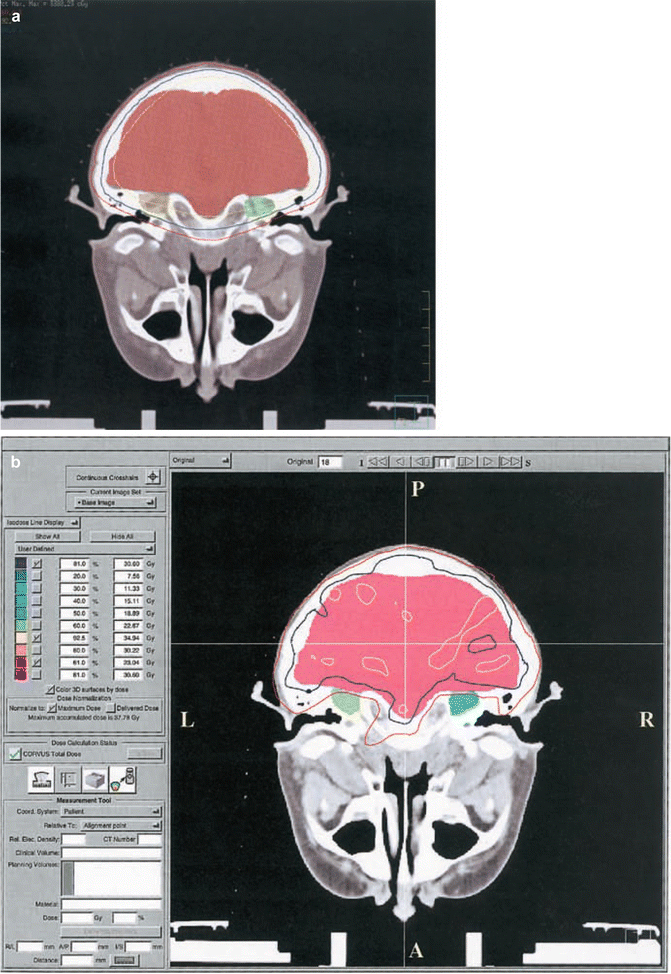
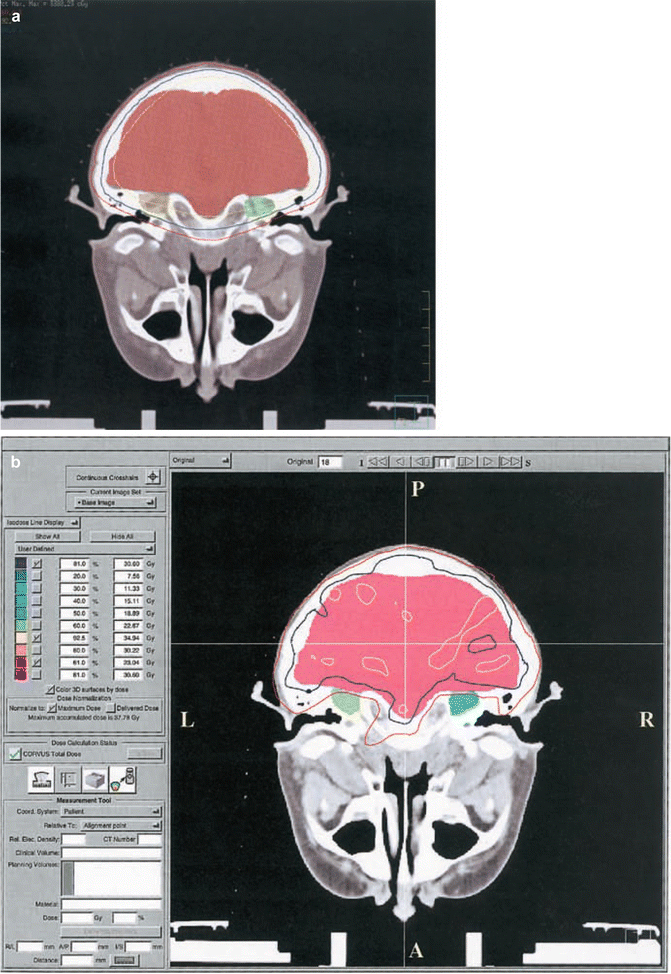
Fig. 16.2
Comparison of axial sections planned with either three-dimensional conformal radiation therapy (3DCRT) (a) or intensity-modulated radiation therapy (IMRT) (b), demonstrating the sparing of inner structures achieved with IMRT without compromising the dose delivered to the target volume
While IMRT has many advantages, there are also disadvantages that should be noted when selecting the treatment modality. With IMRT, the total radiation needed to deliver the plan (i.e., the monitor units) is increased since much of the beam is blocked by MLCs resulting in additional scatter and leakage. Furthermore, with increased beam angles, a larger volume of low dose, or integral dose, is delivered. A study found that, in high-grade gliomas, IMRT actually decreased integral dose by 7–10 % relative to 3DCRT (Hermanto et al. 2007). Furthermore, IMRT results in an inhomogeneous dose over within the treated volume, with hot spots reaching doses of 10–15 % above the prescription dose. Although these hot spots typically fall within the tumor volume, the significance of having regions of extremely high dose is unclear.
To address patient immobilization and daily positioning, some centers use image-guided techniques. These new approaches allow the radiation oncologist to confirm the tumor location every day. One of the most exciting techniques to minimize tumor and patient movement is the use of a CT image of the patient using the “cone-beam” technique that generates an image of the tumor and all surrounding normal structures using the same linear accelerator with which the patient is being treated. Newer machines are commonly incorporating kilovoltage cone-beam imaging, providing images comparable in quality to clinical CT scans, and facilitating accurate image registration. Appropriate adjustments can then be made daily to ensure that the tumor is receiving the prescribed dose of radiation, and normal tissues are receiving doses within their tolerance range.
The emerging concept of image-guided motion management is standard in the radiation oncology community. Motion management techniques primarily center on accounting for respiratory motion. Abdominal compression devices are used to limit the range of motion associated with breathing. Respiratory gating is used to trigger the radiation beam when the target is within a specified window.
16.3.1 Immobilization and Imaging
The initial step of the planning process is patient immobilization, to ensure reliable reproduction of the radiation delivery each day. This position may be different for each patient and depends on the specific location, shape and size of the tumor, and areas at risk for suspected microscopic disease. A variety of customizable immobilization devices are available, including thermoplastic face masks, alpha cradles, and vacuum bags. It is important to note that as the planned course of radiation therapy becomes increasingly conformal and high dose per fraction (often exceeding the tolerance of neighboring critical structures), the importance of reliable and reproducible immobilization becomes critical. Once the patient has been optimally and reproducibly positioned, localization marks are placed on the skin. With the patient in the treatment position, CT images of the area of interest are obtained. These data are then transferred to the planning system, at which point the clinician can define target volumes as well as critical structures. While some well-defined structures can be contoured automatically, most structures must be defined manually. A pretreatment CT scan is generally used for treatment planning, although other imaging modalities such as MRI and PET/CT scans can be co-registered with the CT data.
16.3.2 Target Definition and Planning
The CT images are analyzed jointly by radiation oncologists, by diagnostic radiologists, and occasionally by other treating physicians such as medical oncologists and surgeons. The treatment volume is dictated by the natural history of the tumor. Gross tumor volume (GTV) is defined by physical exam and imaging studies and encompasses the macroscopic extent of the tumor. Clinical target volume (CTV) contains both the GTV and areas at risk for microscopic spread of disease. The planning target volume (PTV) is defined as the CTV surrounded by adequate margin to account for variation in patient position, organ motion, and other movement (Purdy 1999; Hall 2000).
Once target volumes and critical structures are defined, beam geometry and weighting are defined, and dose distribution is calculated (Purdy 1999). As discussed earlier, this can be done using either forward planning where the beam directions and energies are designated by the planner (Fig. 16.3) or inverse planning whereby an optimization algorithm determines the optimal arrangement of beams to deliver dose to the target while respecting the prescribed normal tissue constraints.
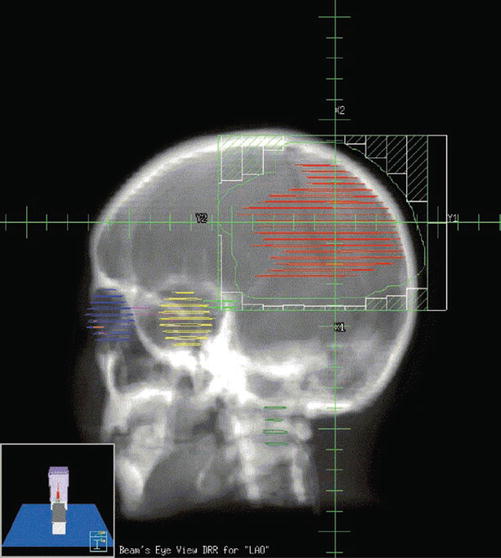
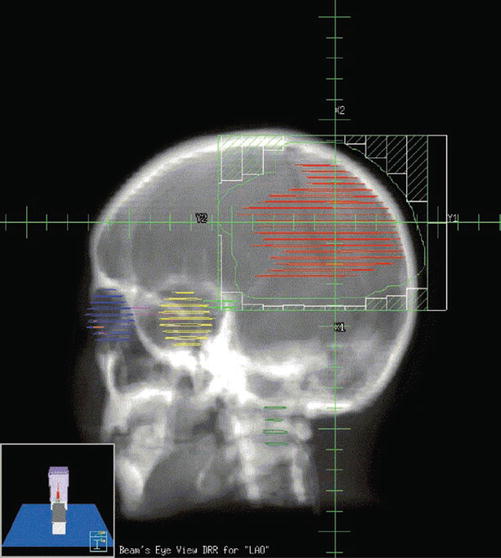
Fig. 16.3
Beam’s eye view (BEV) of a left anterior oblique field in a patient with a supratentorial primitive neuroectodermal tumor (PNET). BEV allows the visualization of the relationship of tumor volumes to those of critical normal tissues, as if looking from the origin of the beam. This allows beam angles and beam shaping to be selected more intelligently. Once an initial plan has been developed, the resulting dose distributions are calculated and evaluated by the clinician. The plan can then be altered to improve on initial results, if necessary. The beam directions as well as their relative weights and shapes are modified to finally optimize the 3DCRT plan (Courtesy of Clayton Akazawa, Department of Radiation Oncology, UCSF)
Plans are then evaluated by viewing isodose curves on serial images of a CT scan (Fig. 16.4a–c), as well as by the generation of dose–volume histograms (DVHs; Fig. 16.5). DVHs can be generated for a tumor volume or other organ of interest, allowing the clinician to evaluate the dose delivered to the total volume. DVHs typically graph percent volume of a given tissue on the Y-axis and dose on the X-axis. This allows a clinician to visualize what percentage of a defined structure is receiving a given dose. These data allow plans to be modified as needed to either increase dose delivered to tumor or decrease dose to a nearby critical structure. Dose constraints are often given prescribing a volume of an organ that cannot exceed a certain dose. For example, a common constraint for normal lung tissue is V20 Gy <20 % which means that the volume receiving 20 Gy should be less than 20 %.
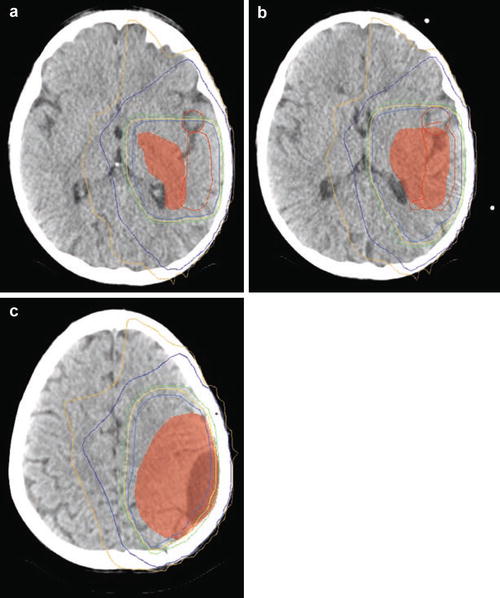
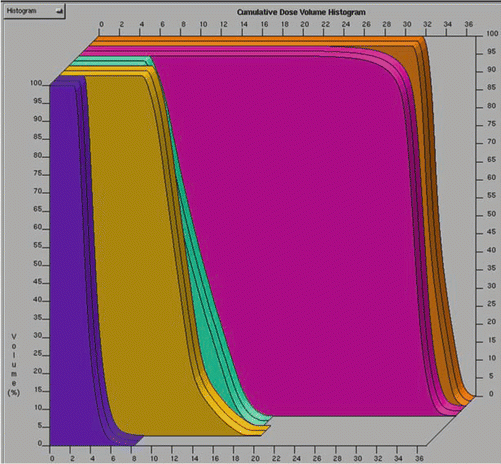
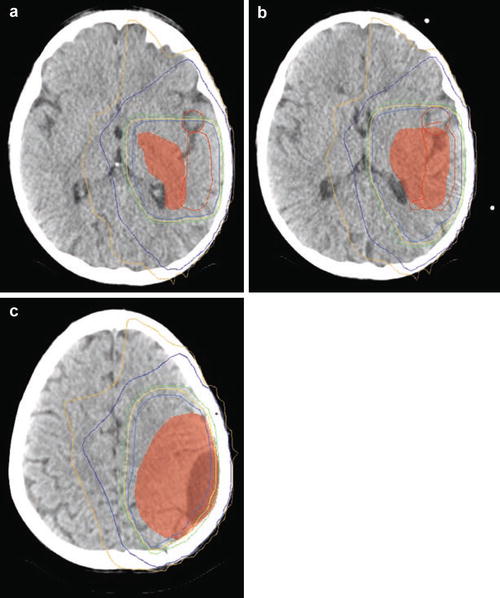
Fig. 16.4
(a–c) Isodose curves of a three-dimensional conformal plan on serial axial slices in a patient with a supratentorial primitive neuroectodermal tumor (PNET). Red line depicts the 99 % isodose line, light blue represents 95 %, yellow depicts 93 %, green depicts 90 %, blue depicts 60 %, and gold depicts 50 % (Courtesy of Clayton Akazawa, Department of Radiation Oncology, UCSF)
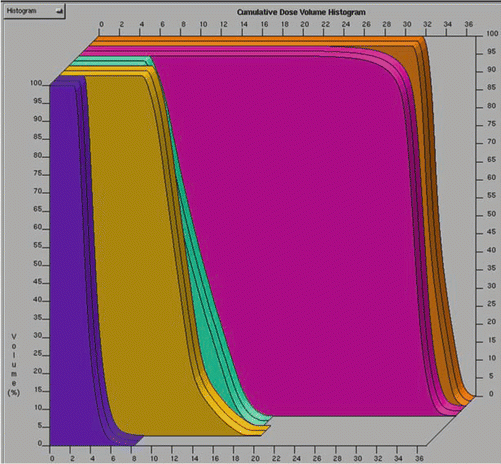
Fig. 16.5
A dose–volume histogram for an intensity-modulated radiation therapy (IMRT) plan in a patient with a medulloblastoma. Clinical target volume (CTV) is depicted in orange, planning target volume (PTV) in pink, left ear in light blue, right ear in orange, and optic chiasm in dark blue (Courtesy of Pam Akazawa, Department of Radiation Oncology, UCSF)
16.3.3 Treatment Verification and Delivery
Treatment verification for IMRT plans is often done using patient-specific QA (quality assurance). This involves delivering the dose to a phantom, embedded with dosimeters, and ensuring that the received radiation agrees with the planned radiation dose. For 3D plans, DRRs corresponding to the planned radiation fields are generated. These DRRs typically display field shapes and tumor volumes, as well as standard radiographic information, such as anatomy.
During the first day of treatment, before the first radiation dose is delivered, the patient position confirmed using a cone-beam CT. For patients undergoing radiation with 3D planning, all the beam angles are confirmed using X-rays from the beam’s eye view, called portal films. These films can be done with conventional portal films or with the use of an electronic portal-imaging device (EPID). Because there is no development time (as there is with conventional portal images), the use of an EPID can significantly shorten the time needed to take portal images of complex multifield plans.
16.4 New Technical Approaches
16.4.1 Charged Particle Therapy (Proton Therapy)
Although proton radiation has similar inherent biological effectiveness as conventional radiation, its charged nature lends it a physical characteristic whereby the dose falls off rapidly (after penetrating a specified distance which is a function of the energy of the particle), often referred to as the “Bragg peak” (Yang 1999). For reference, the dose proximal to the Bragg peak is roughly 30 % of Bragg peak, while the dose afterward is almost zero (Mitin and Zietman 2014). This feature allows the exit dose to be significantly reduced sparing critical structures. It is conventionally accepted that proton beam therapy is superior to standard radiotherapy for skull-base chondrosarcomas and chordomas, but its effectiveness compared to radiosurgery or conformal radiotherapy has yet to be demonstrated in randomized clinical trials. It is often used in posterior fossa tumors as well as craniospinal irradiation, where the target structure is close to the surface of the patient.
The physical advantages of proton beam radiotherapy must be weighed against proton beam contamination by neutrons that may contribute significantly to risks of second malignancies, particularly in children. Additionally, the maturity of radiotherapy planning in protons lags behind photons, often requiring larger margins for proton therapy. A recent review notes an average of 3.5 % + 1 mm for the uncertainty margin when planning with protons (Mitin and Zietman 2014). Improvements in planning software and delivery of protons, particularly with pencil-beam scanners, may allow reduction of the PTV margins as these techonlogies mature.
Despite the lack of Class 1 evidence, retrospective studies do exist to support the use of proton-based radiotherapy in specific subtypes of pediatric intracranial lesions. Macdonald et al. found that in pediatric ependymomas, local control, progression-free survival, and overall survival rates were comparable to those published in the literature for photon radiotherapy. As anticipated, traditional proton therapy and intensity-modulated proton therapy (IMPT) resulted in greater sparing of normal tissue compared to photon-based IMRT (MacDonald et al. 2008). Using a model designed to predict neurocognitive dysfunction after radiation therapy, Merchant et al. concluded that the reduction in lower-dose volumes and mean dose afforded by proton therapy might reduce the incidence of late-term sequelae in children with medulloblastomas, craniopharyngiomas, and optic pathway gliomas (Merchant et al. 2008). Specifically, they found that small, critical, and normal structures such as the cochlea and hypothalamus, which were anatomically separated from the PTV, received substantially less radiation using protons compared with photons. Proton radiotherapy would therefore be expected to reduce the risks of endocrine deficits and hearing loss. In addition, protons lowered the low (0–20 Gy) and intermediate (20–40 Gy) doses to the cerebrum in patients receiving focal radiation. Using longitudinal models of radiation dose–cognitive effects, the data indicated that proton radiotherapy would mitigate intelligence quotient (IQ) loss.
The benefit of proton beam radiotherapy in children is well exemplified by its use for craniospinal irradiation. Comparisons of proton beam, conventional 3D radiation, and IMRT for treatment of the posterior fossa and spinal column suggest superior sparing of normal structures by protons. In particular, protons are likely to mitigate long-term toxicities related to hearing, endocrine, and cardiac functions. For example, 90 % of the cochlea received 101.2 % of posterior fossa boost dose with conventional radiation techniques, 33.4 % with IMRT, and only 2.4 % with protons. Similarly, 50 % of the heart received 72.2 %, 29.5 %, and 0.5 % of the posterior fossa boost dose for conventional X-ray therapy, IMRT, and proton beam therapy, respectively (St. Clair et al. 2004). Comparable reductions in normal tissue doses have been documented in other pediatric disease sites (Lee et al. 2005), such as retinoblastoma and sarcoma.
Recent publications highlight potentially higher toxicities observed after treatment with protons than with photons, and one must acknowledge persistent uncertainties related to proton dose deposition and biological effects. A study examining imaging changes following proton- or photon-based (IMRT) radiotherapy demonstrated more imaging changes following proton-based radiation compared to photon-based IMRT. Furthermore, only proton-treated patients experienced grade 3 or 4 changes and had persistent symptoms, including one grade 5 toxicity related to radiation necrosis documented at autopsy (Gunther et al. 2015). A similar study assessing brainstem toxicity following proton radiotherapy for pediatric brain or skull-base tumors reported a 2-year cumulative incidence of grade 3 or higher brainstem toxicity of 2.1 % ± 0.9 %, with one grade 5 toxicity (Indelicato et al. 2014).
To help assess the proper role of proton beam radiotherapy, the American Society for Radiation Oncology (ASTRO) published the findings of a task force in 2012 (Allen et al. 2012), noting that “In pediatric CNS malignancies PBT appears superior to photon approaches but more data is needed. In large ocular melanomas and chordomas, we believe that there is evidence for a benefit of PBT over photon approaches.” Building on the biological principle of proton therapy, whereby a charged particle with mass can deposit its energy in a small, defined range within the body, carbon therapy is also being explored. Due to the significant cost, it is being investigated only in a few centers in the world.
16.4.2 Intraoperative Radiotherapy
Intraoperative radiotherapy (IORT) typically involves the delivery of a single large fraction of radiation therapy at the time of open surgery (Willett 2001). Radiation is most commonly given to the resection cavity using electrons. Depth of dose is controlled by choice of electron energy and use of bolus. Falloff is rapid beyond the effective range of the selected electron energy. There is little data regarding either the efficacy or the side effect profile of IORT in the treatment of primary pediatric CNS lesions. However, a recent Phase I study using the Photon Radiosurgery System found that IORT to a dose of 10 Gy prescribed to 2-mm depth was feasible and safe; the authors cautioned though that, when dose was maintained but depth increased to 5 mm, side effect, namely, radiation necrosis, increased (Kalapurakal et al. 2006).
16.4.3 Temporary or Permanent Brachytherapy
Interstitial brachytherapy, which involves the placement of radiation sources either directly in tissues or into catheters placed within tissue, allows for the delivery of high doses of radiation to a tumor region. The fundamental difference between brachytherapy and external beam radiation therapy is twofold: (1) the radioactive particle is often an electron in brachytherapy (as opposed to a photon), and (2) the dose falloff is related to geometry and goes as the inverse of the distance squared, since the source is very close to the target (Hall 2000). Both of these features give a sharp dose falloff, allowing highly conformal radiotherapy to be delivered, but, likely, all surgical procedures are highly dependent on operator experience.
Temporary brachytherapy typically entails the implantation of catheters in the tumor or tumor bed, using a variety of radioactive sources, including radium and cesium. In current practice, an 192I source is frequently used, utilizing a robotic remote afterloading system to minimize dose to medical personnel. Permanent brachytherapy implants utilized seeds placed into the tumor cavity, and remain in the cavity, and commonly used sources are 125I and 103Pd.
A retrospective study reported a large series of pediatric brain tumors treated with 125I brachytherapy (Sneed et al. 1996). Twenty-eight children were treated with temporary, high-activity 125I brachytherapy for recurrent or persistent supratentorial, unifocal, well-circumscribed tumors less than 6 cm in diameter that had previously received external beam radiation therapy. Exclusion criteria included tumors with diffuse margins, corpus callosum involvement, or subependymal spread. The most useful result from this study is the documentation of acute and late toxicities. Outcome data, however, are less reliable, given the variety of brain tumors included in the analysis. No Grade III or IV acute or late toxicities occurred. However, 22 patients (79 % of 28 total patients) required at least one reoperation following brachytherapy, and 17 of these 22 patients had evidence of necrosis in the resected specimen.
In an effort to reduce the incidence of radiation necrosis, an additional retrospective pediatric study looked at permanent low-activity 125I seed implants for primary pediatric CNS lesions. Six patients with recurrent disease were enrolled, five of whom had received prior EBRT, and all of whom had reoperations after recurrence; only two patients had local failures at the first site of recurrence, leading the authors to conclude that low-activity permanent 125I seed implants can help to provide good local control while diminishing the risk of significant treatment-related morbidity (Rostomily et al. 2001).
16.4.4 Stereotactic Radiosurgery (SBS) and Stereotactic Radiotherapy (SRT)
The primary difference with SRS and SRT over conventionally fractionated approaches is that the goal is to ablate the target region (analogous to surgical excision). This requires several factors: (1) excellent imaging capable of precisely delineating the tumor volume and neighboring critical structures for accurate targeting, (2) immobilization and intrafraction imaging to ensure stability of the target, and (3) motion management strategies to target moving tumors (i.e., lung and liver). Conceptually, SRS and SBRT consist of multiple beams of radiation, all converging at the designated target volume. The large number of noncoplanar beams allows increased conformality over the limited number of angles that a standard linear accelerator is capable of, translating into a sharper dose falloff outside of the target region. CyberKnife radiotherapy can be used to achieve high conformality to the tumor, while only a low dose is given to the surrounding brain tissue. In this example shown in Fig. 16.6, the green line represents 23 % of the dose, where the red represents the prescription isodose line (70 %).
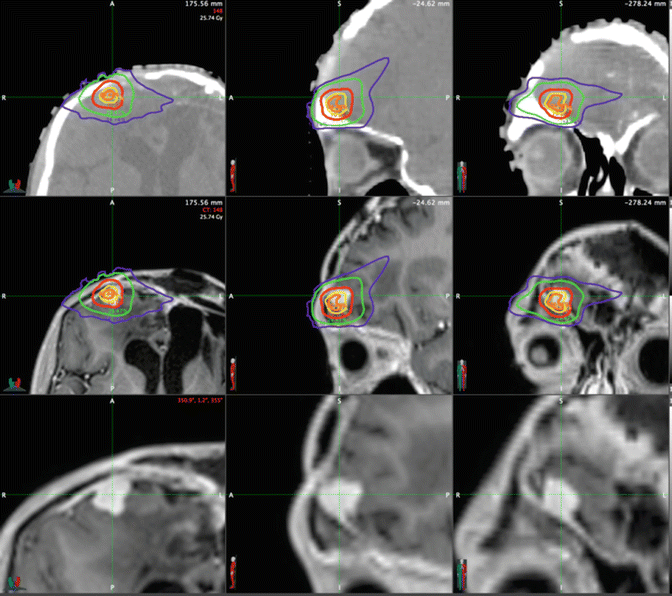
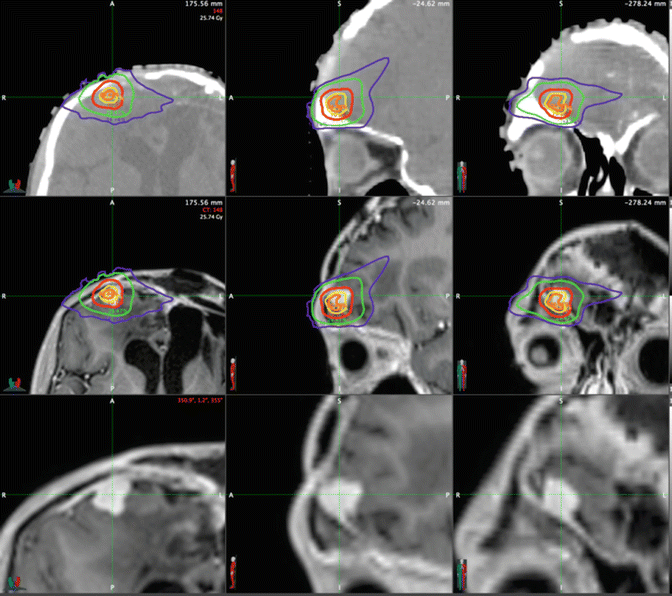
Fig. 16.6
SBRT used to treat a 16-year-old male with history of malignant neuroglial tumor with numerous recurrences, following multiple resections, chemotherapy trials, and multiple radiation treatments including SBRT to two lesions in the RUL and extensive radiation to the brain with a single fraction of SBRT via CyberKnife to a left frontal recurrence with 19 Gy in 1 fraction to the 70 % isodose line (red isodose curve). The top panel contains the planning CT scan, the middle panel has the MRI (post-gadolinium volume) with dosimetry overlaid, and the bottom shows the zoomed in tumor volume without isodose lines (Courtesy Dr. Jean Nakamura, UC San Francisco, Department of Radiation Oncology)
There are several instruments capable of providing SBRT, and most new linear accelerators have this capability. The first instrument used for SRS was the Gamma Knife® with 201 collimated beams of cobalt-60 radiation. In this modality, a patient’s head is physically immobilized to the table and a single or multidose (<≤5) regiment of focused radiation to a small intracranial target. Frequently, sensitive normal structures lie near the target volume. Rapid falloff of radiation dose outside the target spares adjacent normal tissues and maintains a safe, acceptable level of irradiation. The value of SRS has been demonstrated by many retrospective studies, which include a variety of benign and malignant brain tumors (Kondziolka et al. 2000).
Radiosurgery use in pediatric populations is less frequent; however, retrospective case series have proven its feasibility. One of the few outcome studies retrospectively examined a population of 90 pediatric patients, the majority of whom were diagnosed with medulloblastoma, anaplastic astrocytoma, glioblastoma, or primitive neuroectodermal tumor (PNET). In some patients, SRS was used as an initial treatment, while in others it was implemented at the time of recurrence. The study confirmed the safety of SRS in pediatric patients, with a minimal side effect profile; there was an apparent reduction in local failure at the site of first recurrence compared to historical controls, and efficacy was increased when SRS was used as initial management rather than as salvage. The authors concluded that, in cases where residual or recurrent tumor is focally unresectable, an SRS boost might be beneficial (Hodgson et al. 2001).
Stereotactic radiotherapy (SRT) uses a noninvasive immobilization device and fractionates dose. The number of fractions is typically five or less, but this is an arbitrary definition based on US billing codes. Again, the literature is lacking in randomized controlled trials, but a prospective trial studied the effect of SRT in 50 pediatric patients with low-grade astrocytomas, including optic pathway gliomas. All the children were treated with SRT for progression following either surgery or chemotherapy. The total dose delivered was 52.2 Gy in 1.8 Gy daily fractions. Overall survival was 97.8 % at 5 years and 82 % at 8 years. Because tight margins were used in the hope of reducing late sequelae, there was some concern over marginal failures, but none were observed within a median follow-up of 6.9 years. The excellent local control observed supports the use of SRT for small, localized, low-grade CNS lesions (Marcus et al. 2005).
16.4.5 Neutron Beam Therapy
Neutrons, a particle with mass but no charge, deposit their dose more densely than conventional photon radiation, and the increased relative biological effect results in greater cell death. In addition, hypoxic cells exhibit less resistance to neutron radiation than to conventional photon radiation. Although clinical trials of neutron beam therapy in the treatment of malignant gliomas have resulted in a higher rate of tumor control than treatment with photons, no improvement in survival was demonstrated, likely due to increased necrosis associated with neutron therapy (Battermann 1980; Catteral et al. 1980; Griffin et al. 1983; Laramore et al. 1988). In a randomized study examining the optimal dose of neutrons for a limited-volume neutron boost combined with photon whole-brain radiotherapy, no beneficial combination was documented (Laramore et al. 1988).
16.4.6 Boron Neutron Capture Therapy
Boron neutron capture therapy (BNCT) was first proposed in 1936, but has yet to make significant clinical inroads and is included here for completeness. A stable isotope of boron, 10B, is administered to patients in a pharmacologic preparation and accumulates in tumor cells. Normal and tumor tissues are then irradiated by broad-beam, low-energy thermal or epithermal neutron irradiation. The 10B nuclei have a high probability of thermal neutron capture that results in nuclear fission. High LET particles are created with a range of only one cell diameter, killing only the cells in the immediate vicinity of the boron compound.
The clinical utility of BNCT depends on developing new 10B-containing compounds that accumulate more selectively within tumor cells and achieve higher concentrations in these cells than within blood, scalp, and normal brain tissues (Diaz et al. 2000). The major compound used in clinical trials to date, p-boronophenylalanine (BPA), produces 10B concentrations 3.5-fold higher in tumor and 1.5-fold higher in scalp than in blood (Chadha et al. 1998). Recent preclinical research in a rat model used a boronated monoclonal antibody (L8A4) directed against EGFRvIII, a mutant form of the EGF receptor. The authors found a statistically significant increase in overall survival when compared to intravenous BPA; the combination of intravenous BPA and boronated L8A4 proved still more potent, leading to an increase in overall survival greater than that achieved with either therapy alone (Yang et al. 2008).
16.5 Toxicity of Radiation Therapy
A wide range of potential toxicities complicates the implementation of radiation therapy in the treatment of tumors of the craniospinal axis. These toxicities can be severe and debilitating, particularly in pediatric patients (Donahue 1992; Syndikus et al. 1994; Kalapurakal and Thomas 1997). Care should be taken to minimize these effects. Treatment of intracranial tumors can result in damage to the eye, ear, brain, and hypothalamic–pituitary axis, as well as impairment of normal growth. Treatment of the spine can result in growth deficits and damage to the spinal cord. Specific potential acute side effects of radiation to the central nervous system include epilation, skin reactions, otitis, hematopoietic depression, and somnolence. Specific late toxicities of radiation include radionecrosis, myelopathy, leukoencephalopathy, vascular injury, neuropsychologic sequelae, endocrine dysfunction, bone and tooth abnormalities, ocular complications, ototoxicity, and induction of second primary tumors (Donahue 1992; Syndikus et al. 1994; Kalapurakal and Thomas 1997). Table 16.1 delineates the radiation doses associated with late toxicities that may result from radiation therapy to the CNS.
Table 16.1
Late toxicities of CNS irradiation
Structure | Late effect | Threshold dose (Gy) |
---|---|---|
Conventional fractionation | ||
Spinal cord | Chronic progressive myelitis | 45 |
Brain | Radiation necrosis | 60 |
Intellectual deficits | 12–18 | |
Eye | ||
Lens | Cataract formation | 8 |
Retina | Radiation retinopathy | 45 |
Optic nerve | Optic neuritis | 50 |
Inner ear | Sensorineural hearing loss | 40–50 |
16.5.1 Spinal Cord
Although rare, severe damage to the spinal cord can result following radiation therapy, with transection of the cord at the affected level being the most severe potential consequence. This usually takes the form of a chronic progressive myelitis. Wara et al. reported a 1 % incidence of spinal cord damage at 42 Gy and a 5 % incidence at 45 Gy. A number of reports have indicated that tolerance of the cervical spinal cord to radiation toxicity is somewhat higher than 45 Gy in adults (Wara et al. 1975). However, it is unclear what the cervical spinal cord tolerance is in pediatric patients. Radiation to the spinal cord can also result in Lhermitte’s syndrome, which is characterized by tingling, numbness, and a sensation of electric shock. Symptoms are often present only with neck flexion. Lhermitte’s syndrome is typically self-limiting, presenting within the first 1–3 months following radiation, and having an average duration of 3–4 months. Craniospinal radiation can result in decreased truncal, or sitting, height. This is due to decreased growth of the vertebral bodies following radiation therapy and becomes clinically evident at doses greater than 20 Gy.
16.5.2 Brain
Acute reactions during radiation therapy, thought to result from disruption of the blood–brain barrier, are uncommon. However, there are reports of edema following single conventional fractions (Kramer and Lee 1974). Clinically, apparent acute changes are more common with hypofractionated doses, such as those used in radiosurgery (Loeffler et al. 1990). Steroids can be administered to address edema.
Subacute reactions are more common and are thought to be due to transient demyelination (Boldrey and Sheline 1966). These effects typically occur within the first few months following radiation and usually resolve within 6–9 months. Delayed, transitory clinical manifestations of radiation, including somnolence syndrome, are seen in a large number of patients receiving prophylactic craniospinal radiation along with intrathecal chemotherapy for acute lymphoblastic lymphoma (ALL) (Littman et al. 1984). Severe subacute effects such as rapidly progressive ataxia are rare and are generally associated with fractions larger than 2.0 Gy and total doses larger than 50 Gy (Lampert and Alegria 1964).
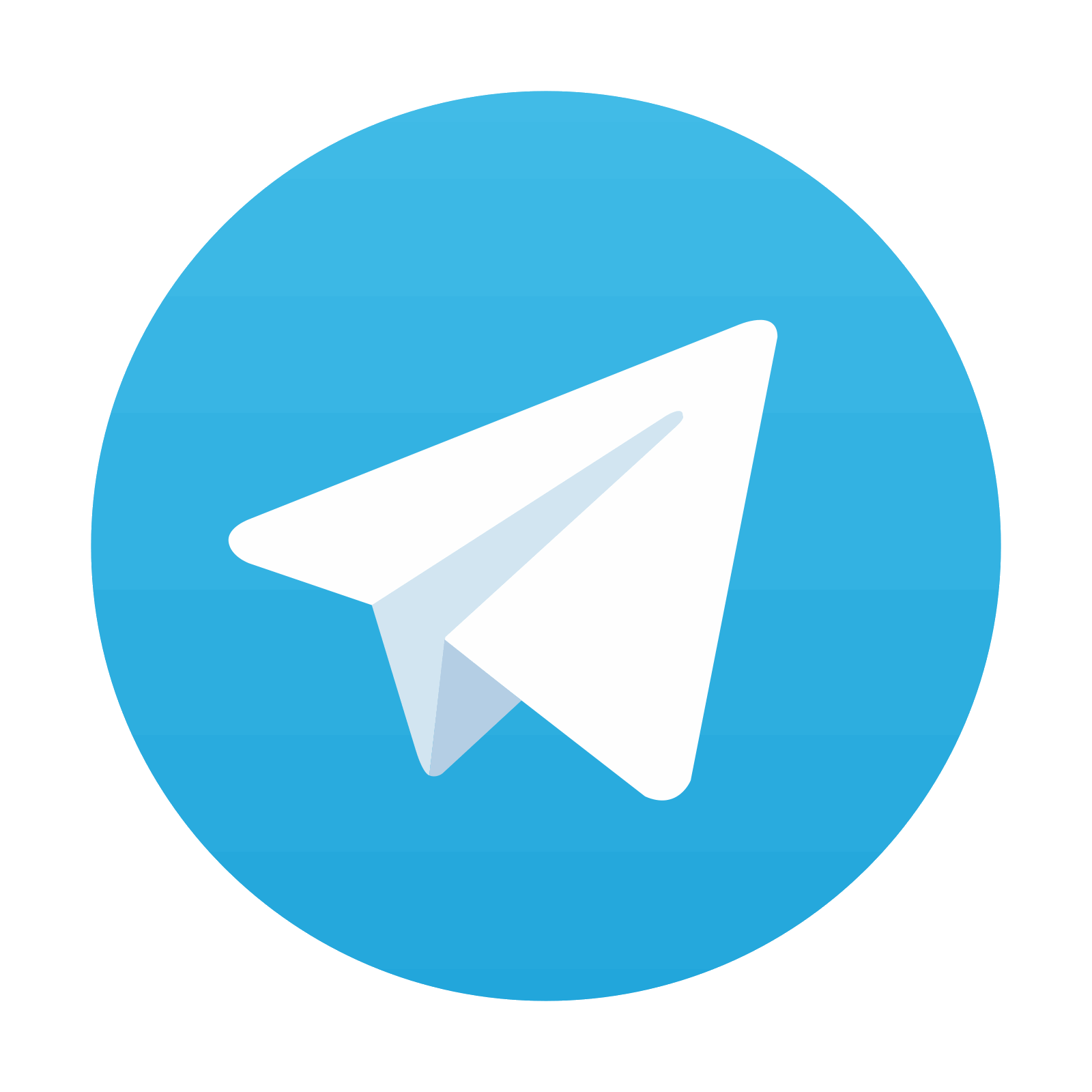
Stay updated, free articles. Join our Telegram channel
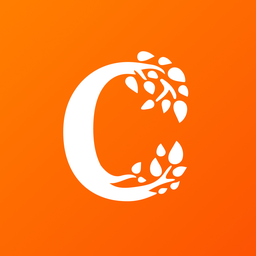
Full access? Get Clinical Tree
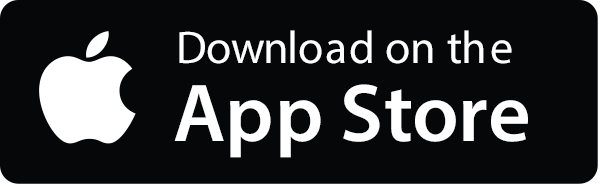
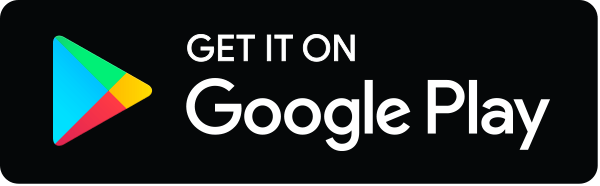