Biology of Childhood Cancer
Christopher Denny
Daniel S. Wechsler
Kathleen M. Sakamoto
INTRODUCTION
The age-old axiom that pediatric patients are not simply small adults with disease definitely holds true in oncology. Even when viewed from the broadest perspective, there are fundamental differences between childhood and adult malignancies. First, the incidence of cancer in children is only a fraction of that seen in the adult population. Second, although there is some overlap, children and adults develop different types of cancer. The epithelium-derived tumors that form the majority of adult cancer are rarely seen in children. Even when adult and child have the same malignancy by histologic appearance, they may not be equivalent on a biologic level. For example, pediatric patients with pre-B acute lymphoblastic leukemia (ALL) as a group respond very well to current therapy whereas adults with clinically the same disease fare much worse.
These clinical observations suggest that biology and the pathogenesis of childhood and adult malignancies are different. One feature that sets the pediatric and adult populations apart is that children are in the process of normal growth and development. At a cellular level, an enormously complex program of division and differentiation is being played out. In this developing physiologic milieu, the potential may exist for oncogenic transformation to occur in particular cell populations that are either less vulnerable or not present in a mature adult. This may in part account for the age-related peaks seen with certain pediatric cancers. In a simplistic way, some pediatric cancers may start as errors in normal development.
Current effective treatments for the vast majority of pediatric cancers are triumphs of empiric trial design. Although many viable regimens have been developed, it is not always clear why they are effective (e.g., l-asparaginase in ALL and platinum compounds in germ cell neoplasms). From a pragmatic perspective, many pediatric patients continue to benefit from empirically derived regimens. However, when these regimens fall short and the cancer returns, there is usually little insight as to why treatment failed.
Therapies based on the biology of a particular tumor may not only prove to be more effective but just as importantly provide a logical framework in which to work. The expectation is that inhibiting specific molecular pathways that cancer cells need for their continued growth will have greater efficacy with fewer deleterious side effects than is seen with current cytotoxic regimens. Should therapeutic resistance be encountered, follow-up testing could be performed to determine whether the drug is sufficiently inhibiting its molecular target.
In this chapter, we hope to highlight those biologic concepts and molecular pathways that both play dominant roles in oncogenesis and hold the most potential for therapeutic intervention. We present this first from a molecular perspective involving individual cells and end on a more macro level looking at the interactions among cell populations. This is not a rigid schema, and cross talk across levels is more the rule than the exception.
SIGNAL TRANSDUCTION
Overview
In multicellular organisms, cells have developed finely regulated molecular systems that allow them to respond to environmental cues and to effectively communicate with one another. These systems begin to function at the very earliest stages of embryogenesis and play critical roles in normal growth and development. In adults, signaling modules are the cell-based components that are primarily responsible for maintaining physiologic homeostasis within tissues and organ systems.
Cell signaling systems have evolved to fulfill three basic requirements. First, because speed is of the essence, most signaling systems rely on enzymatic alterations of component proteins that can be performed in seconds. Site-selective phosphorylation of tyrosine, serine, and threonine residues on signaling proteins by protein kinases is a common way that this is accomplished. These posttranslational modifications promote assembly of multiprotein heteromeric complexes with other signaling components like pieces to a jigsaw puzzle. Just as quickly as this system can be activated, it can be turned off through the action of specific protein phosphorylases that return signaling molecules to their dephosphorylated baseline state.
Second, systems must be able to resolve multiple inputs. This is accomplished through use of cascading structures. Ligand-receptor interactions initiate signals at the cell surface that are then propagated through the cytoplasm through a series of interconnected molecular cascades. As a result, multiple distinct ligand-receptor events can be integrated into a cohesive signal. Finally, cell signaling systems must be sufficiently robust so that variation of individual signaling components has minimal effect on the result. This is accomplished through extensive cross talk among signaling pathways as well as feed forward and feedback loops. It now appears that these systems behave as integrated components within a cell signaling network.
With such exquisite control over cell behavior, it is not surprising that signal transduction systems are frequently subverted in the process of oncogenesis. Because so much of signal transduction is governed enzymatically, these systems are at least partially accessible to pharmacologic intervention. For this reason, many of the targeted anticancer agents being pioneered today focus on modulating signal transduction pathways.
Receptor Tyrosine Kinases
Receptor tyrosine kinases (RTKs) are a major class of cell surface receptors that initiate signaling cascades (Fig. 4.1). RTKs are constructed in modular fashion and are composed of distinct domains that can be functionally delineated from each other. A typical RTK consists of an extracellular ligand-binding domain followed by a single hydrophobic transmembrane region that is linked to an intracellular protein kinase domain. Binding of the ligand induces RTK dimerization and/or conformational changes that serve to activate their intracellular protein kinases, resulting in autophosphorylation at specific tyrosine residues within the RTK. These modifications provide binding surfaces to which intracellular signaling molecules dock and can in turn be phosphorylated by the activated RTK.
Even at this level, there is inherent complexity built into the system. Within each RTK family, there can be both multiple RTK members and multiple ligands. For example, in the fibroblast growth factor (FGF) family there are more than 18 ligands that can
bind to four different FGF receptors.1 If coexpressed in the same cell, different RTKs from the same family may heterodimerize, resulting in activation of signaling pathways that can differ from those activated by their respective homodimers.
bind to four different FGF receptors.1 If coexpressed in the same cell, different RTKs from the same family may heterodimerize, resulting in activation of signaling pathways that can differ from those activated by their respective homodimers.
Since their discovery, RTKs have been implicated in playing important biologic roles in wide variety of human malignancies. Instances where specific RTKs are mutated in a particular cancer argue strongly for their direct involvement in oncogenesis. Gene amplifications leading to overexpression of ErbB2 and PDGFR have been described in subpopulations of patients with breast cancer and glioblastoma multiforme (GBM), respectively (for reviews, see 2,3). Similarly activating point mutations and internal deletions give rise to mutant forms of c-KIT and EGFR in patients with gastrointestinal stromal tumor (GIST) and non-small cell lung carcinoma.4,5 Finally, RTK structure can be altered through tumor-associated chromosomal translocations that fuse a portion of the RTK to an unrelated gene, resulting in expression of chimeric proteins.6,7,8,9 In most of these fusions, the extracellular ligand-binding domains of the parent RTKs are replaced by polypeptide sequences that promote receptor dimerization in the absence of ligand (Fig. 4.1). In effect, these oncogenic fusions behave as RTKs that are in a constant state of activation.
RAS/MAPK Pathway Perturbation in Myeloid Leukemia
Activated RTKs transmit their signal into the cell by recruiting intermediary signaling proteins and potentially activating them through phosphorylation. This initiates a cascade in which these activated intermediate molecules in turn phosphorylate other signaling proteins to further propagate the signal. Over the last few decades, the number of molecularly characterized signaling pathways has grown dramatically as has an appreciation for how they can be subverted during oncogenesis through somatic mutation.10 A particularly compelling example is provided by the RAS/MAPK pathway and myeloid leukemias (for review, see Ref. 11).
K-, H-, and NRAS are closely related members of a much larger family of protein GTPases (for review, see Ref. 12). RAS proteins exist in dynamic equilibrium between a GTP-bound active state and a GDP-bound inactive state. The conversion between these two states is catalyzed by specific regulatory molecules. RAS GAPs (GTPase activating proteins) accelerate hydrolysis of bound GTP to GDP and inactivate RAS. RAS GEFs (guanine nucleotide exchange factors) promote the replacement of RAS GDP with GTP and activate RAS. Physiologic activation of many RTKs by ligand binding typically leads to recruitment and activation of numerous signaling molecules including SOS, an RAS GEF, which shifts the equilibrium toward activated RAS (Fig. 4.1). GTP-bound RAS then is able to bind and activate a number of effector molecules, a major one being RAF, a serine/threonine protein kinase.13 This initiates a cascade involving sequential activation of protein kinases through phosphorylation, leading to changes in gene expression that promote cellular proliferation.
Though activating mutations in RAS have been found in a variety of adult tumors, it seems that the RAS/MAPK pathway has been systematically targeted in myeloid leukemias (for review, see Ref. 14). Starting at the cell surface, mutations of the FLT3 or c-KIT receptors are found in 30% of acute myelogenous leukemia (AML) patients, which result in unregulated activation of these RTKs. The BCR/ABL fusion, which is present in tumor cells of virtually all chronic myelogenous leukemia (CML) patients, directly recruits SOS, resulting in RAS activation. In juvenile myelomonocytic leukemia (JMML), loss-of-function mutations in the NF1 gene, which encodes a primary RAS GAP, shifts the equilibrium
in favor of activated RAS. Finally specific activating mutations of RAS itself have been found in patients with AML, JMML, and chronic myelomonocytic leukemia (CMML).
in favor of activated RAS. Finally specific activating mutations of RAS itself have been found in patients with AML, JMML, and chronic myelomonocytic leukemia (CMML).
Aberrant Signaling in Brain Tumors
The signaling module regulated by phosphatidylinositol-3-kinases (PI3Ks) is frequently targeted in CNS as well as other cancers (Fig. 4.2). The numerous PI3Ks have been parsed into three different families on the basis of structure and function. Type 1A PI3K can be activated by direct interaction with activated RTKs or through signaling intermediates such as RAS (for review, see Ref. 7). Such interactions induce a conformational change in PI3K and bring it in close proximity to the inner plasma membrane. Here PI3K catalyzes the conversion of lipid-bound phosphatidylinositol(4,5)phosphate (PIP2) to phosphatylinositol(3,4,5)phosphate (PIP3). The local increase in PIP3 level provides a platform for the PDK1 protein kinase to phosphorylate its primary target, the AKT family of serine/threonine kinases. Once activated, AKT then phosphorylates a number of effector molecules that promote entry into cell cycle and inhibit programmed cell death.
Perhaps the most intensively studied AKT effector is mTOR, the catalytic component of the multiprotein complex, mTORC1.15 MTOR is a highly conserved protein kinase whose normal function is to regulate the cell’s response to growth factor and nutrient exposures. Phospho-AKT, both directly and through the RAS-like G-protein RHEB, derepresses the mTORC1 multiprotein complex. Activation of mTORC1 in turn upregulates metabolic pathways important for cell growth such as ribosomal biogenesis and enhancement of mRNA translation.
The primary off switch to the PI3K signaling pathway is the lipid phosphatase PTEN, which catalyzes the dephosphorylation of PIP3 reverting it back to PIP2. Lacking a surface to which they can dock, PDK1 and AKT cease to interact resulting in the eventual dephosphorylation and inactivation of AKT. Since PTEN is a nonredundant component in this pathway, if it is lost or mutated, there are no other proteins that can fulfill the same regulatory function.
Given its unique role, it is not surprising that somatic deletion or loss of function of PTEN is one of the most common mutations found in human cancers.16 A particularly compelling association is found in human brain tumors where a high proportion of secondary glioblastoma multiforme tumors (GBMs) harbor activating EGFR and/or loss-of-function PTEN mutations.17 It now appears that clinical response to EGFR inhibitors may hinge on whether a particular GBM has one or both mutations.18 Patients whose tumors harbored both mutations generally did not respond to EGFR inhibition, suggesting that disruption of PTEN can serve as a mechanism of resistance.
Mutation of the Hedgehog signaling pathway is another potential mechanism in the oncogenesis of brain tumors. This canonical pathway functions by sequential derepression of its components. Upon Hedgehog binding to its cell surface receptor (PTCH1), Smoothened (SMO) is liberated and promotes the disassociation of the GLI1/2 transcription factors from their inhibitor, SUFO (for reviews, see 19,20). GLI1/2 are then free to traverse to the nucleus and initiate a transcriptional program promoting cell growth. Germline loss-of-function mutations of PTCH1 are seen in patients with Gorlin syndrome who are at high risk for developing aggressive basal cell skin carcinomas as well as medulloblastomas. Even more compelling, about 30% tumors from patients with spontaneous medulloblastoma exhibit transcriptional profiles consistent with upregulation of Hedgehog signaling.21 Discrete mutations in Hedgehog signaling components are found in about half of these tumors.
Therapeutic Strategies
The dominant role that aberrant signaling can have in driving tumor cell growth has stimulated much activity toward developing molecular inhibitors to oncogenic pathway components. By any measure, the BCR/ABL fusion in CML has proven to be the seminal example of how such therapeutic strategies can be successfully applied. Imatinib, the first BCR/ABL antagonist, was a congener of a small molecule inhibitor of PDGFR. Imatinib’s clinical potential was demonstrated early on when it was found to inhibit growth of primary bone marrow cultures from CML patients by almost two orders of magnitude greater than normal bone marrow.22 Clinical trials have proved equally promising. The 7-year event-free survival for chronic-phase CML patients treated with imatinib is 81%.23
Although imatinib has revolutionized the treatment of CML, with time, 17% of patients who had achieved complete cytogenic
response suffer relapse. In a majority of cases, molecular analyses of these tumors reveal clonal outgrowths of leukemia cells harboring BCR/ABL fusions with mutations that prevent imatinib binding.24 Recognizing these mutations has allowed for structure-based rational design of potent next-generation BCR/ABL inhibitors including dasatinib and nilotinib that are in various stages of clinical evaluation.25,26,27
response suffer relapse. In a majority of cases, molecular analyses of these tumors reveal clonal outgrowths of leukemia cells harboring BCR/ABL fusions with mutations that prevent imatinib binding.24 Recognizing these mutations has allowed for structure-based rational design of potent next-generation BCR/ABL inhibitors including dasatinib and nilotinib that are in various stages of clinical evaluation.25,26,27
Though targeting BCR/ABL has led to a paradigm shift in the therapeutic approach to CML, trying to apply a similar strategy to other malignancies has yielded more modest success. It is not that there is a dearth of potential therapeutic targets. High-throughput genomics technologies have identified tumor-associated activating mutations in a wide variety of “druggable” signal transduction molecules. Biochemically effective pharmacologic inhibitors have been devised for many of these proteins. However, clinical responses have been neither as profound nor as durable as those seen with BCR/ABL tyrosine kinase inhibitors in CML.
In some instances, the primary mechanism of resistance can be outgrowth of a subpopulation of genetically modified cells that produce a further mutated or an increased quantity of the targeted signal transduction molecule. In effect, the tumor cells break through the blockade imposed by the targeted therapy. For example, 57% of glioblastomas exhibit EGFR upregulation via activating mutation and/or genomic amplification. Exposure of glioblastoma cells to lapatinib, an EGFR tyrosine kinase inhibitor, can lead to a dynamic increase in the copy number and expression of the EGFR gene, thereby outcompeting its inhibitor.28
The most prevalent resistance mechanism to targeted inhibition of signal transduction molecules may be one of adaptation: tumor cells find a way around the roadblock by utilizing alternative pathways. For example, tumor cells from some glioblastoma patients who developed lapatinib-resistant disease demonstrated compensatory upregulation of the PDGFRβ RTK.29 Similarly, the rapid development of clinical resistance to IGF1R inhibition in Ewing sarcoma patients may at least in part be due to secondary utilization of the canonical insulin signal transduction pathway.30 In a variation on this theme, AML cells harboring mutated FLT3 RTKs can evade the biologic effects of FLT3 small molecule inhibitors by secondary upregulation of its cognate FLT3 ligand.31,32
These mechanisms of developing acquired resistance are not mutually exclusive and can be viewed as consequences of two central themes in tumor biology: (a) individual tumors are composed of a heterogeneous collection of malignant cells with differing genetic and epigenetic features; (b) signal transduction pathways are extensively interconnected and dynamic systems. Under certain circumstances, cell responses depend not only on pathways that are activated but also on the timing of the signaling stimuli. These two precepts suggest that combination strategies that target multiple signaling pathways may prove more efficacious.33
Recently, new technologies have been developed that can assess signal transduction pathway responses at the single-cell level in a highly parallel fashion.34,35 Determining the variation in signaling response simultaneously across many thousands of cells has not only led to functional definition of subpopulations of cells within a tumor, but also to a clearer picture of how these signaling networks operate.36 These approaches, coupled with innovative mathematical models, hold promise that strategies for identifying promising therapeutic combinations will be forthcoming in the near future.37,38
GENE EXPRESSION
Overview
If cancer cells are responding to intrinsic albeit aberrant signals, then regulation of gene expression acts as the final arbiter. Signaling cascades detect and integrate extracellular cues that are then transmitted to the nucleus and result in modulating the expression of specific sets of genes. The ability to simultaneously sequence the human genome and assess global mRNA expression has provided new insight into the depth and complexity of transcriptional programs. Though cytoplasmic signaling pathways can behave as relatively compact modules, transcription programs frequently resemble highly branched nonhierarchical networks.39 It is the sum of these gene networks that in large part dictate cellular behavior. For this reason, specifically and effectively reversing transcriptional programs that drive cancer cells poses a significant therapeutic challenge.
Transcription
Many genes are regulated at the point of initiation of transcription (Fig. 4.3). Two events need to occur in order for transcription of a gene to start. First, the surrounding chromatin must be put in a molecular conformation that will allow transcription. This is accomplished by proteins that modify the DNA itself as well as histones, DNA-binding proteins that form higher-order chromatin structure. Second, large multiprotein complexes need to be recruited to the core promoters that are located just upstream of the transcription start sites. These complexes include general transcription factors (GTFs) that contain the RNA polymerase II holoenzyme (RNAPII), as well as coactivator or corepressor complexes. At some genes, these two events occur simultaneously.
Transcriptional activators are key regulators of the transcription process. These proteins act as links between DNA sequences and basal transcription complex proteins. Transcriptional activators bind to DNA regulatory sequences, usually located within a gene locus, and enhance the recruitment and/or function of transcription complexes. Gene regulatory sequences typically encode binding sites for many different activators. Furthermore, occupancy by multiple activators is usually necessary for upregulating transcription at a particular locus. This combinatorial requirement allows for specific regulation of a large number of genes by a relatively small number of individual transcriptional activators.
Most transcriptional activators are modular in structure, with domains that mediate site-specific binding to particular DNA sequences and separate regions that mediate specific protein-protein interactions. Changes in the primary sequences of these domains can have functional consequences due to alteration of protein-DNA or protein-protein specificity, resulting in altered expression of target genes. This is precisely what is thought to happen in certain tumor-associated mutations found in particular pediatric cancers (see in the following section).
De-Regulating the Regulators—MYC
Given their integral roles in regulating normal gene expression, it is not surprising that transcriptional activators and repressors are potential oncogenic targets. In fact, inappropriate expression of transcriptional regulators can profoundly affect normal cell growth and differentiation, and many have been implicated in oncogenesis.
Misexpression of a transcriptional regulator resulting from a chromosomal abnormality provides strong evidence that it plays a causative role in oncogenesis. The MYC family of transcription factors is a compelling example (for reviews, see 40,41,42). MYC, a tightly regulated basic-helix-loop-helix-leucine-zipper (bHLHZip) protein, binds to DNA by heterodimerizing with MAX, a ubiquitously expressed bHLHZip member (Fig. 4.4). MYC requires MAX in order to bind to DNA at specific sequences within MYC target genes. MYC can then interact with general transcription factors (GTFs) and recruit coactivator complexes (e.g., CBP/p300) that result in modulation of target gene expression. MYC can act as a transcriptional activator or repressor depending on the target gene and the proteins with which it interacts.
A large number of candidate MYC target genes has been identified and characterized (for review, see 43,44). Analyses in several model systems reveal a consistent pattern of upregulation of genes promoting cell cycle progression (CDK4, cyclins D1, D2, B1), ribosome biosynthesis (rDNA, RNA polymerase III), and metabolism (CAD, ODC). Simultaneously, cell cycle checkpoint regulators (p15INK4A, p21) are downregulated along with genes promoting cell adhesion. This repertoire of gene expression changes promotes growth, mitosis, and enhanced motility, features found in aggressive tumors.
Given these profound effects on cell behavior, it is not surprising that the MYC family is normally under tight regulatory control at both transcriptional and functional level. The bHLHZip proteins MNT and MXD1 through MXD4 (formerly MAD1-4) bind to DNA as MAX heterodimers. However, unlike MYC, they recruit corepressor complexes that downregulate target gene expression. Not only do MNT and MXDs compete with MYC for limited amounts of MAX, but they also actively antagonize MYC-mediated biologic effects. Altering the MYC-MNT/MXD equilibrium could thus serve as a powerful oncogenic stimulus.
This is in fact what happens in a number of pediatric malignancies. In Burkitt lymphoma, tumor-associated chromosomal translocations juxtapose immunoglobulin regulatory elements to the cMYC gene (see Chapter 3). As a result, the cMYC protein is inappropriately expressed, promoting abnormal B-lymphocyte growth. Mouse models with immunoglobulin-MYC transgenes develop B-cell malignancies, consistent with cMYC playing a causative role. Gene amplification can also serve to deregulate MYC family members. The finding that MYCN amplification confers a very poor prognosis in pediatric patients with neuroblastoma is indicative of its importance in the biology of this tumor. The frequent finding of an increase in copy number in the region of chromosome 8p, which encodes cMYC, suggests that misexpression of MYC genes is a common theme in many human cancers.
Transcription Factor Chimerism
Hundreds of chromosomal translocations have been associated with specific malignancies (see Chapter 3). Though there are many potential molecular consequences of such genomic rearrangements, the most common outcome is the direct merging of two normally distinct genes. This results in the expression of chimeric transcripts and proteins that correspond to the portions of each partner gene that have been fused into a single molecule. In most cancer-related gene fusions, at least one partner is frequently found to be involved in transcriptional regulation. These chimeric proteins retain some of the activities of their normal partners but also acquire new functionality that can promote oncogenesis.
A prime example of a pediatric malignancy-associated transcription factor chimera is EWS-FLI1. The t(11;22)(q24;q12) chromosomal translocation gives rise to EWS-FLI1 in 85% to 90% of Ewing sarcomas.45 EWS is a member of the FET family of RNA-binding proteins, and the portion of EWS in the fusion contains a strong transcriptional activation domain. FLI1 is an ETS family transcription factor and contributes a DNA-binding domain. The EWS-FLI1 fusion acts as a potent oncogene: its expression results in both activation and repression of a series of target genes that are critical to oncogenesis.46 The ability of EWS-FLI1 to modulate a whole network of downstream effector gene targets—some direct and some indirect—contributes to achieving the various hallmarks of oncogenesis. It should also be noted that there is heterogeneity within EWS-FLI1 translocations (there are several different breakpoints in the EWS and FLI1 genes), and that translocations of EWS and other ETS family members
(EWS-ERG is seen in 10% of Ewing sarcoma) result in similar patterns of gene expression.45
(EWS-ERG is seen in 10% of Ewing sarcoma) result in similar patterns of gene expression.45
Similarly, the signature genetic change in pediatric alveolar rhabdomyosarcoma (aRMS) is the PAX3-FOXO1 fusion gene that derives from the t(2;13)(q35;q14) translocation.47,48 PAX3-FOXO1 is seen in 55% of aRMS cases and fuses the amino terminal paired and homeobox domains of PAX3 to the transcriptional activation domain of FOXO1 (FKHR). A similar translocation (t(1;13) (p36;q14)) fuses PAX7 to FOXO1 in 22% of aRMS. Both PAX3 and PAX7 are expressed during normal muscle development; the presence of PAX3/7-FOXO1 interferes with normal skeletal muscle differentiation and results in tumorigenesis. While PAX3/7-FOXO1 is critical, its presence alone is not sufficient to induce transformation—other genetic alterations (MYCN amplification, deregulation of IGF2 and FGFR4) are required for tumors to develop. A large number of PAX3/7-FOXO1 target genes has been identified, and many of these have been studied in vitro and in vivo.48 A better understanding of the complex interplay among PAX3/7-FOXO1 and its targets and cooperating genes will hopefully lead to novel treatment approaches for aRMS.
Epigenetic Regulation
The ability to modulate gene expression independent of nucleotide sequence is the purview of epigenetics.49,50 Epigenetic modifications result in remodeling of chromatin—the delicately balanced complex of DNA with specific histone proteins that allows DNA to be compacted into the cell nucleus. Chromatin remodeling may in turn lead to altered (increased/decreased) gene expression as specific genomic sequences become more or less accessible to regulatory transcription factors. Modification of DNA (by site-specific methylation at CpG sites) or of histones (mostly by methylation and acetylation but also by phosphorylation and ubiquitination) can affect the interaction of DNA with histones and ultimately affect gene expression. This DNA-histone interaction results from specific covalent marks on both DNA and histones that are regulated by families of enzymes (see below). Disruption of the “normal” DNA-histone interaction can then lead to perturbed gene expression that can promote tumor cell growth and survival. Indeed, cancer cells frequently exhibit dramatically modified DNA and histone modification patterns that are associated with tumorigenesis and tumor progression.51,52
Modifications of DNA and histones are carried out by specific enzymes: covalent marks are applied by “writers” (acetylases, methylases, phosphorylases), recognized by “readers” (bromodomains, chromodomians, PHD fingers, WD40 repeats) and removed by “erasers” (deacetylases, demethylases, phosphatases) (for review, see 49,50,53). The normal function of these modifying enzymes may be altered as a result of somatic mutation or chromosomal translocations that either enhance or impair activity. Thus, miswriting, misreading, and miserasing of these marks is strongly associated with oncogenesis and tumor progression.53 The importance of perturbed epigenetic modification of histones in oncogenesis54 is highlighted by the following specific examples:
The MLL gene (chromosome 11q23) is involved in recurrent translocations in many pediatric (especially infant) and treatment-related leukemias.55 The MLL protein, a component of several chromatin remodeling protein complexes, methylates histone H3 at the lysine 4 position (H3K4). While H3K4 trimethylation results in efficient transcription of target genes, the carboxy terminal histone methyltransferase (HMT) domain is lost in all MLL translocations. Through mechanisms that are poorly understood, but at least in part dependent on specific partners, leukemogenesis then occurs.
EZH2 is an HMT that plays an essential role in embryonic development and stem cell renewal. EZH2, a catalytic subunit of the polycomb repressive complex 2 (PRC2), specifically methylates H3K27 and leads to its trimethylation; deregulated EZH2 activity contributes to oncogenesis.56 EZH2 is overexpressed in a variety of solid tumors, and recurrent inactivating mutations have also been identified in the domain that is required for its HMT activity. Regulation by EZH2 is complex and context dependent: altered activity of “eraser” H3K27 histone demethylases (HDM—e.g., JMJD3 and UTX) may modulate the effects of EZH2.
A critical role for histone modification in pediatric gliomas has recently been delineated.57 Several groups58,59,60,61 have determined that 80% of pediatric diffuse intrinsic pontine gliomas (DIPGs) harbor lysine → methionine (K27M) mutations in histone H3.3 or H3.1. The precise mechanism by which K27M mutations result in gliomagenesis is unclear, since these mutations affect only a small fraction of the total H3 protein in a cell. However, it is believed that the K27M mutation results in a gain of function, whereby the K27M mutant histone inhibits the PRC2 complex at very low concentrations, thereby reducing global H3K27 trimethylation; this in turn promotes DIPG formation.
DOT1L is an HMT that specifically methylates H3K79. DOT1L interacts with AF10, a partner for both MLL and PI-CALM in leukemogenic fusions, and its mistargeting to HOXA gene loci results in hypermethylation and upregulation of HOXA expression. This, in turn, contributes to leukemogenesis.
Histone acetylation, mediated by several families of histone acetyl transferases (HATs) including CBP/p300, is highly associated with transcriptional activation. Deregulated HAT activity correlates with oncogenesis, but as with many epigenetic modifiers, both loss and activation of HATs can be oncogenic.49 In contrast to HATs, histone deacetylases (HDACs) generally facilitate transcriptional repression. When HDACs are recruited to tumor suppressor gene loci, their expression is repressed and increased proliferation can result.
The posttranslational modification of histones by methylation, acetylation, and phosphorylation support the notion of a “histone code” that plays a key role in regulating the dynamics of chromatin.62 According to this hypothesis, distinct combinations of covalent posttranslational modifications of histones influence chromatin structure and lead to varied transcriptional outputs.63 The term “histone onco-modification” has been proposed to describe the changes in histones that are important in the development of cancer.56 The examples above represent only a glimpse into the histone modifications that have been described, highlighting the complexity of regulation and pointing toward potential difficulties of therapeutically intervening with histone modification.
RNA Processing
Gene regulation does not only occur at the level of transcription initiation (Fig. 4.3). A typical mammalian gene is made up of a linear array of exons that encode peptide sequences, interspersed with relatively long noncoding introns. As genes are transcribed, introns are spliced out of nascent transcripts in the process of forming mature mRNAs. The molecular machinery that is responsible for recognizing genomic intron-exon boundaries and accurately processing them rivals the transcriptional regulatory system in complexity. Not surprisingly, there is flexibility built into this system. Variability in intron splicing can result in a single gene giving rise to mRNA isoforms with different exon composition and that correspondingly encode structurally different polypeptides.
It is estimated that alternative exon splicing occurs in upward of 75% of human genes.64 Given the ubiquity of this genetic process, it is tempting to postulate that mutation of either splicing factors or genomic intron-exon boundaries could play a dominant role in human disease, and in some cases this appears to be true (for review, see Ref. 65). Indeed alternative splicing is now considered to play an active role in human cancer.66,67 It is clear that transcripts encoding functionally different isoforms of key regulatory proteins
are found in certain malignancies (for review, see Ref. 68). For example, alternatively spliced forms of HOXA9 have been described in a subset of leukemias. While HOXA genes clearly play a role in leukemogenesis, this newly identified regulatory feature adds complexity to understanding how HOXA genes specifically contribute to leukemia development.
are found in certain malignancies (for review, see Ref. 68). For example, alternatively spliced forms of HOXA9 have been described in a subset of leukemias. While HOXA genes clearly play a role in leukemogenesis, this newly identified regulatory feature adds complexity to understanding how HOXA genes specifically contribute to leukemia development.
Posttrancriptional Regulation
Even after a mature mRNA is formed and exported to the cytoplasm, there are additional layers of control. Adaptable cellular systems regulate transcript half-life in the cell and modulate how efficiently its encoded sequence is translated into protein. Of these regulatory systems, none is more powerful or pervasive than the heterogenous group of processes mediated through small noncoding RNAs (see 69,70). Within this group, microRNAs (miRNAs) play potentially dominant roles in human oncogenesis.
Almost two decades ago, while trying to develop an efficient genetic tool that would permit knockdown of specific gene expression, Fire and Mello discovered RNA interference (RNAi) in the worm Caenorhabditis elegans.71 They found that any transcript could be targeted for destruction by simply transducing into cells a double-stranded RNA with the same nucleotide sequence as the target. The remarkable sensitivity and specificity of RNAi could not be accounted for by previously described mechanisms. This suggested that RNAi was being mediated by a new physiologic system whose normal purpose was gene silencing.
There has subsequently been an explosion of information regarding how miRNAs are formed and the highly conserved mechanisms through which they regulate mRNA half-life and translation into protein. In mammals, most miRNAs are encoded within the introns of genes (Fig. 4.5). As these genes are transcribed and their introns spliced out, hairpin-looped miRNAs are excised by the Drosha enzyme complex and exported to the cytoplasm. There, miRNAs are further cleaved by the Dicer endonuclease and incorporated into the multiprotein RISC complex as short ˜22 bp single-stranded RNAs. It is the sequence of this incorporated RNA that specifies both which mRNAs are targeted by the RISC complex and the molecular consequences of the interaction. Transcripts with regions of perfect complementarity to the guide sequence are cleaved and rapidly degraded. Transcripts that have only partial homology are not degraded but are inefficiently translated into protein. Most endogenous mammalian miRNAs appear to work primarily through this latter mode of translation inhibition.
Estimates of just how many endogenous miRNAs exist in our genome vary from hundreds to thousands.72 Because miRNAs can target transcripts to which they have only partial nucleotide sequence identity, trying to predict which miRNAs modulate the expression of specific genes has been difficult. There are, however, numerous examples of specific miRNAs that modulate the expression of key regulatory genes involved in a wide variety of cellular processes including signal transduction, cell cycle control, differentiation, and apoptosis.73,74
As with transcription factors, the question of how miRNAs actively participate in the process of human oncogenesis is under active investigation.75,76,77 High-throughput expression studies have found extensive differences in miRNA expression profiles between normal and cancer cells. In fact, miRNA expression signatures provide an accurate means of classifying human tumors, even cancers of unknown primaries.78 The finding that certain miRNA genomic clusters are deleted or amplified in particular human malignancies more directly reinforces the notion that misregulation of miRNAs could play an active role in oncogenesis.79,80 Moreover, manipulation of miRNAs may have potential therapeutic applications (for review, see 81,82).
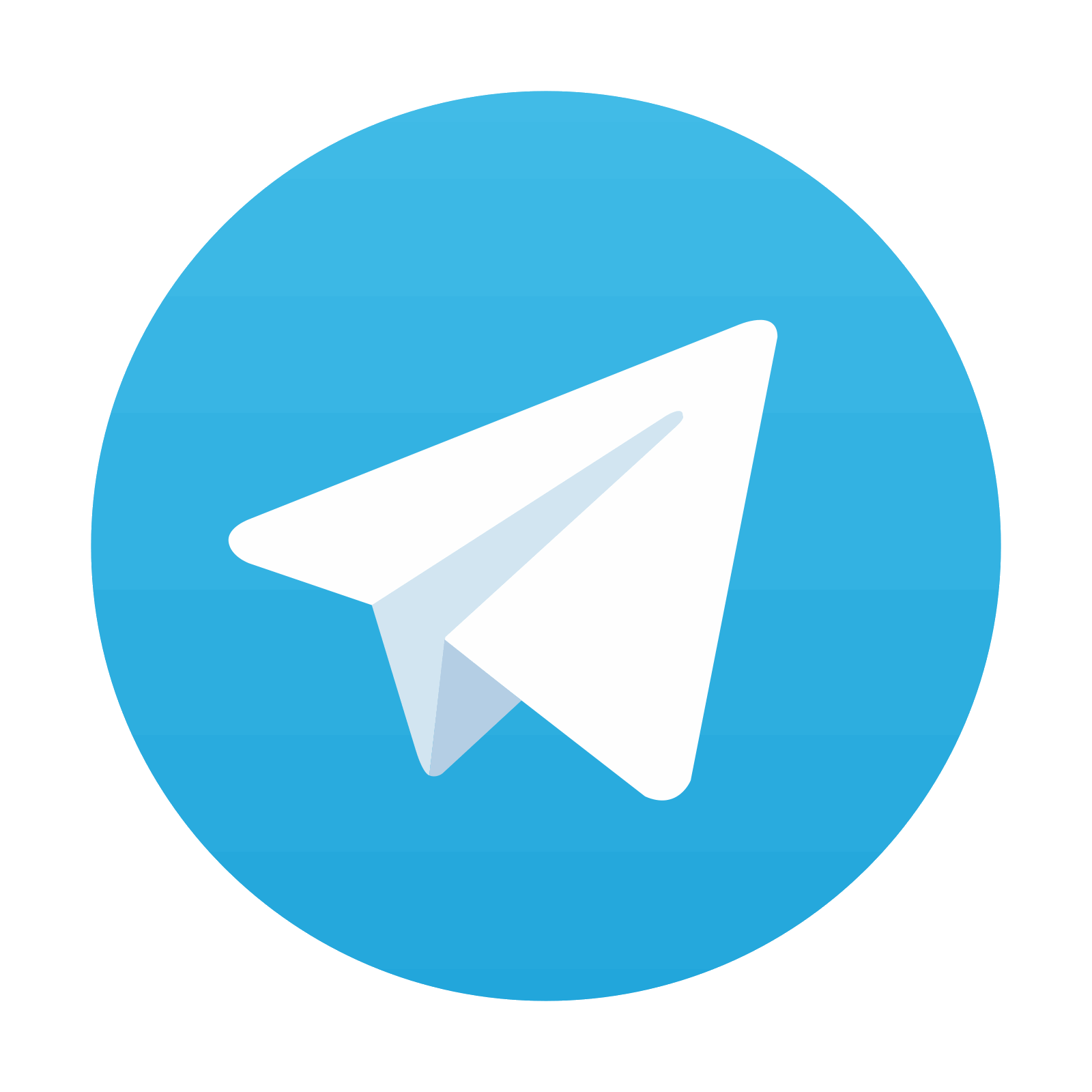
Stay updated, free articles. Join our Telegram channel
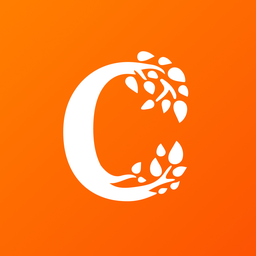
Full access? Get Clinical Tree
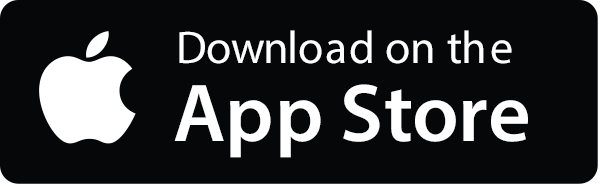
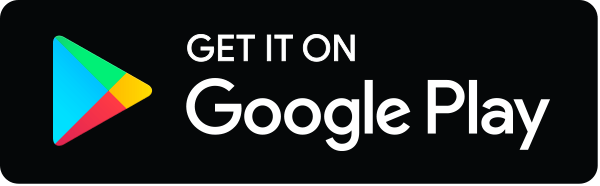