11.1 Introduction
Vitamin A deficiency is the most common cause of childhood blindness, causing 250 000–500 000 children to go blind every year, half of whom will die within the year. Approximately another 150 million are at increased risk of dying in childhood from infectious diseases owing to an inadequate vitamin A status. Vitamin A is a generic term used for a group of structurally related chemical compounds known as retinoids that possess qualitatively the biological activity of retinol. Although an essential nutrient needed in only small amounts, it is necessary for normal functioning of a large number of regulatory and other physiological processes of the human body. Vitamin A deficiency disorders occur when body reserves are depleted to the limit at which physiological functions are impaired, even if there is no evidence of clinical xerophthalmia (the pathological eye signs of vitamin A deficiency).
Under normal physiological conditions, nearly 90% of the stored vitamin is found in the liver. Depletion of stored vitamin A is usually seen as the consequence of an inadequate dietary intake of the vitamin itself over a period of time, although losses are increased by associated infection. Vitamin A is extensively recycled between the plasma, liver and other tissues, and the rate of utilization by specific tissues can show some adaptation to diminishing availability. This homeostatic adaptation and recycling serves to maintain a relatively constant level in the blood until body stores become depleted below a critical point.
Sources of vitamin A
Vitamin A in the diets of most human communities comes from a very wide variety of plant and animal sources in order to meet their daily requirements. In the more industrialized countries over two-thirds of dietary vitamin A is derived from animal sources as preformed vitamin A, whereas in the developing world, communities depend primarily on provitamin A carotenoids from plant sources. These latter countries are at higher risk of vitamin A deficiency in their populations, especially where rice is the staple and there is poverty. Common dietary sources of preformed vitamin A are liver, milk and milk products, eggs and fish. The richest sources are liver oils of fish, such as the shark, halibut and cod, and of marine mammals, such as the polar bear. In marine fish, vitamin A1 alcohol (retinol) is the storage form of vitamin A, while in freshwater fish it is the vitamin A2 alcohol (3-dehydroretinol), having only about 40% of the activity of retinol. The livers of ox, sheep, calves or chicken also contain vitamin A at concentrations comparable to cod liver oil. Eggs, milk and other dairy products, such as butter and cheese, are all moderate sources. Meat, such as beef, mutton and pork, is a relatively poor source of preformed vitamin A.
Provitamin A carotenoids are found in many plant foods such as yellow and orange fruit and vegetables, and in dark green leafy vegetables such as amaranth and spinach, although the color of fruit and vegetables is not necessarily an indicator of the concentration of provitamin A. Yellow fruits such as papaya, mango and orange, and vegetables such as carrots, yellow pumpkin, orange sweet potato and yellow cassava have appreciable amounts of provitamin A carotenoids. In the tomato, the major constituent is lycopene, a nutritionally inactive pigment. Red palm oil is the richest natural source of carotenoids. Cereal grains, especially when milled, are a poor source.
Bioavailability
Preformed vitamin A from animal foods, which is primarily present in the diet as retinyl esters, is generally assumed to be 70–90% bioavailable when consumed in usual amounts. Bioavailability, which includes absorption and bioconversion, of provitamin A carotenoids in food is much less, depending on the food, preparation, cooking methods and so on. To reflect this substantially lower biological activity of provitamin A carotenoids in foods in the human diet, the current commonly used unit for quantifying vitamin A activity in foods is the retinol equivalent (RE), which is basically 1 μg of all-trans-retinol. The current useage is that 6 μg of β-carotene or 12 μg of other all-trans-carotenoids in foods is equivalent to 1 μg of vitamin A activity or 1 RE. The total RE value for a given food is calculated by adding the actual amount of retinol to the adjusted equivalent amounts of the provitamin A carotenoids present in the food. The efficiency rate of conversion varies between one-quarter and one-tenth depending on the amount of β-carotene in a meal. It has been set at one-twelfth for all other vitamin A precursors, but this is currently being questioned. Further, all-trans carotenoids have more provitamin A activity than the cis isomers. The conversion process is more efficient in vitamin A-deficient individuals and less efficient in those who are vitamin A replete. However, this conversion ratio is a gross average and recently suggested conversion ratios of carotene to vitamin A in the body range from 26:1 for carrots and green leafy vegetables to 12:1 for fruits.
Evidence indicates that the bioavailability of dietary carotenoids is affected by a large number of factors, including the chemical nature, amount ingested and preexisting nutrient status. The matrix in which a carotenoid is embedded in a particular food appears to be an important determinant of its bioavailability. In green leaves, carotenoids are present within chloro-plasts as pigment–protein complexes, whereas in some vegetables and fruits, carotenoids are found in lipid droplets. Cooking can greatly reduce the matrix effect and thus help in the release of carotenoids, but prolonged heating may lead to oxidative degradation of carotenoids. The absorption of carotenoids depends not only on sufficient fat in the diet, but also on sufficient protein and zinc; while a large amount of dietary fiber such as pectin is known to reduce the bioavailability of carotenoids. Systemic or parasitic infection reduces carotenoid bioavailability, and is thus of greater significance in developing countries, where the parasite infection rates may be high. All these factors need to be taken into account when assessing the bioavailability of carotene from various dietary sources. Therefore using dietary tables to estimate the apparent adequacy of dietary intake of vitamin A in the form of carotenoids does not necessarily indicate adequate status in a population.
In the past, the amount of vitamin A was expressed in international units (IU), a system still sometimes used in food composition tables and particularly in labeling vitamin A supplements. One IU equals 0.3 μg of retinol, 0.344 μg of retinyl acetate and 0.55 μg of retinyl palmitate, all in their all-trans isomers. One IU of all-trans-β-carotene is defined as 0.6 μg. Vitamin A concentrations in tissues, including serum and breast milk for analysis, are now expressed in molar terms to conform to the Systeme Internationale (SI units). In SI units, the conversion factor for 1 μg retinol is equal to 0.003491 μmol per liter of fluid or per gram of tissue.
Chemistry and metabolism of vitamin A
all-trans retinol is the parent substance of the vitamin A group. Retinol (vitamin A1 alcohol) consists of a substituted cyclohexene (β-ionone) ring, a tetraene side-chain and a primary hydroxyl group at C15. The hydroxyl group of retinol may be esterified with a fatty acid to form retinyl ester, a major storage form in the body. Retinal (retinaldehyde) is formed by the oxidation of the hydroxyl group of retinol in the body, which may be further oxidized to a carboxylic acid, retinoic acid. In nature, retinol and its metabolites have a number of geometric isomers with specific physiological functions, one of which is 11-cis-retinaldehyde, which has a role in vision.
Of over 600 carotenoids that have been isolated from nature, only 50 possess the biological activity of vitamin A, and these are known as provitamin A carotenoids. Of these, the most active and quantitatively important provitamin A in foodstuffs is all-trans-β-carotene. For provitamin A activity, carotenoids must contain at least one β-ionone ring that is not hydroxylated and a polyene side-chain. Because of the large number (usually nine to 13) of conjugated double bonds, each carotenoid can form many geometric isomers. For both vitamin A and provitamin A carotenoids, the all-trans isomers are the most nutritionally active forms; cis isomers show about half, or even less, of the activity of the all-trans isomers.
Vitamin A and carotenoids are insoluble in water but are readily miscible with most organic solvents. Because of the structural conjugated double bonds, these compounds when dissolved in solution are sensitive to isomerization, oxidation and polymerization, particularly in the presence of light, oxygen and high temperature. This has important implications for storage of the vitamin in health centers and warehouses. Vitamin A in its natural form, whether present in liver or bound to protein in the plasma, is stable when stored frozen in the dark at a temperature of – 70°C. Carotenoids present in the serum or tissue are also stable at – 70°C in the absence of light under argon, but tend to be more sensitive than vitamin A to destruction. This stability is important when serum samples are being collected during surveys for later analysis. Vitamin A and carotenoids show a characteristic absorption spectrum, which is used in identification and quantification of vitamin A and carotenoids in biological samples.
Vitamin A and carotenoids are released from protein in the stomach by proteolysis, and then aggregate with other lipids and pass into the small intestine. In the presence of bile salts, essentially all retinyl esters and some carotenoids (xanthophylls) are hydrolyzed, primarily by an enzyme located in the brush border of intestinal mucosal cells and by the pancreatic esterases. The resulting unesterified retinol, xanthophylls and provitamin A carotenoids, in micellar form, are absorbed by the intestinal mucosal cells. Within the mucosal cells, provitamin A carotenoids are oxidatively cleaved by a specific 15,15′-dioxygenase enzyme to form retinal, which binds to a cellular retinol binding protein (CRBP type II), and then is reduced to retinol by retinal reductase. In the mucosal cells, retinol is ester-ified by two different enzyme systems: lecithin: retinol acyltransferase and acyl-coenzyme A (acyl-CoA) retinol transferase.
Nearly all of the absorbed forms of vitamin A, as retinyl esters with a small amount of retinol, hydrocarbon carotenoids and xanthophylls, are then incorporated into chylomicrons that are rapidly transported from enterocytes via the lymphatics to the systemic circulation. In the circulation, the chylomicrons are hydrolyzed by lipoprotein lipase to form chylomicron remnants, which then clear all vitamin A, including carotenoids, into the liver.
About 90% of the total vitamin A in the body, mainly as retinyl palmitate, is stored in the liver of well-nourished individuals. Vitamin A from the liver store is released into the circulation as retinol bound to a specific transport protein synthesized in liver, retinol binding protein, (RBP) at a ratio of 1:1 to form a complex (holo-RBP). The holo-RBP in the plasma forms a complex with another protein, transthyretin. This holo-RBP-transthyretin complex is then transported to various target tissues for utilization.
11.2 Consequences of vitamin A deficiency
The public health significance of vitamin A deficiency has been redefined since the 1970s, to include its likely association with the deaths of over one million young children each year worldwide. Between 125 and 250 million preschool-aged children are at risk of being vitamin A deficient, with high-risk populations located mostly in the poorer periequatorial regions of the world (see Figure 11.1 and Section 11.3). It also remains the most common cause of preventable childhood blindness, causing tragic and unnecessary loss of vision in young children. This clinical manifestation (xerophthalmia) reflects likely deficiency in the wider community from which such affected children come. It is now recognized as a condition also affecting women, especially when pregnant or lactating.
Figure 11.1 The global prevalence of vitamin A deficiency. Source: WHO/UNICEF/IVACG (1995a). Reproduced with permission from the WHO.
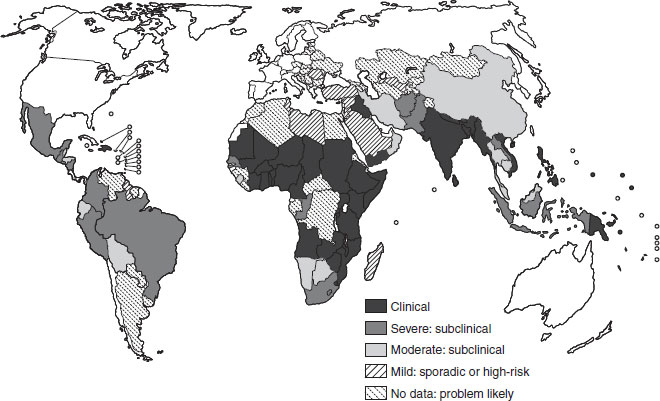
Histopathological and physiological effects
The varied expression of vitamin A deficiency represents the many bodily tissues and systems that are affected by a deficiency of the vitamin. While the main impact appears to be on the integrity of the epithelial tissues, there is also involvement of the enzymic, autoimmune, gene regulation and vision systems.
Much of the histopathological information is derived from animal studies, although with some important differences between animals’ and humans’ systems; for example, the impact on the respiratory system. In the rat, the sequence of the widespread keratinizing metaplasia that follows vitamin A deficiency occurs earliest in the genitourinary tract, then the respiratory tract and then the alimentary tract. Despite eye signs having long defined the deficiency syndrome, the histological changes in the eye actually follow those of the respiratory tract. Atrophy and hypoplasia of glandular ducts usually precede the keratinization of the epithelium, with extreme atrophy of the thymus being universal. Infection commonly follows secondary to the metaplastic changes, suggesting that a keratinized surface provides a conducive substrate for bacterial replication. There are also significant reductions in goblet cell density and secretory granules before any change in the number of ciliated epithelial cells.
In general, the clinical manifestations of vitamin A deficiency depend more on the function of the epithelial surfaces affected than on a functional impact on a particular organ. For example, the dryness of the eye in xerophthalmia is caused by the keratinizing metaplasia of the epithelial tissue of the conjunctiva of the eye, whereas diarrhea is likely to be due to the effect on the lining of the bowel. Nevertheless, the physiological and clinical effects will reflect the many functions of vitamin A in the body, namely, on vision, maintenance of epithelia and immune function. Retinoic acid has also been shown to have a major function in the regulation of gene expression and tissue differentiation.
Clinical and pathological effects
The clinical effects of vitamin A deficiency that are best recognized and described are those affecting the eye (xerophthalmia) and which will lead to irreversible blindness if the deficiency is not corrected, unless death intervenes first. However, as described above, vitamin A deficiency affects virtually all the mucous membranes of the body, and the impact on the eye is later than that on some other organs. These underlying mechanisms are now recognized to affect reductions in immuno-competence, as well as other physiological changes. These lead to increased morbidity and mortality, probably throughout the life cycle, but usually described in infants and young children, and more recently women of reproductive age. The effects are seen most markedly in periods of greatest need: early growth, pregnancy and lactation, and when infections are occurring at the same time. The eye signs making up xerophthalmia will be considered first; then the impact of vitamin A on immunocompetence and infection, morbidity and growth, the associations of vitamin A with malnutrition, and finally its impact on mortality.
Xerophthalmia and keratomalacia
Vitamin A deficiency has long been defined in terms of clinical eye signs, which actually represent a rather more severe manifestation of the deficiency. Xerophthalmia (from the Greek xeros, drying, and ophthalmia, of the eye) represents the ocular consequences of vitamin A deficiency that include night blindness (XN), conjunctival xerosis (X1A), Bitot’s spots (X1B), corneal xerosis (X2), ulceration (X3A) or necrosis/keratomalacia (X3B). In the early 1980s, it was estimated that half a million children developed corneal xerophthalmia each year in south and south-east Asia alone, but these estimates have improved because of decreasing trends in the incidence of corneal xerophthalmia since the 1980s in these regions, so that the current estimate of global occurrence of corneal disease is likely to be around 500 000 per year at most. Even so, vitamin A deficiency remains the most common preventable cause of childhood blindness in the world. Approximately half of all corneal cases lead to blindness and of these blinded children, half will die within the year.
Conjunctival and corneal xerosis, corneal ulceration and necrosis, and retinal dysfunction leading to poor dark adaptation and night blindness comprise the ocular consequences of vitamin A deficiency. A full description of clinical signs with colored illustrations can be found in the World Health Organization (WHO) guide to vitamin A (WHO, 1995b).
Night blindness (XN)
Night blindness is the earliest specific clinical symptom of vitamin A deficiency and is usually the most prevalent stage of xerophthalmia. Its occurrence reflects a failure in rod photoreceptor cells in the retina to maintain peripheral vision under dimly lit conditions. Opsin, a protein, binds covalently with 11-cis-retinal to form rhodopsin (visual purple). Light exposure, even at very low levels, to the back of the eye bleaches rhodopsin. This reaction initiates an electrochemical impulse along the optic nerve to the brain that results in vision. A visual cycle is completed when the vitamin A aldehyde is returned to rods in the outer segment to form rhodopsin. Lack of vitamin A disables this cycle, resulting in poor vision in dim light that, if sufficiently severe, results in night blindness. Typically, a history of night blindness can be elicited using a local term for the condition, often translated as “chicken eyes” (chickens lack rods and, thus, night vision) or “twilight” or “evening” blindness.
A history of night blindness is associated with low-to-deficient serum retinol concentrations in preschool-aged children and pregnant women. Night-blind young children and mothers stumble in poor visibility and stay inactive at dusk and at night. Other nutritional and disease conditions that could contribute to night blindness (e.g. zinc deficiency, wasting, anemia, infection) may be more prevalent in south Asia than in southeast Asia or Africa, where the condition is described less often. Night blindness condition is more readily ascertained in pregnant women than in children for both reasons of reporting and physiology, and may therefore be a better indicator of vitamin A deficiency than by using reports from young children, although there are other, far less common, causes of night blindness in pregnancy besides vitamin A deficiency.
Conjunctival xerosis (X1A) with Bitot’s spots (X1B)
The keratinizing metaplasia effect on mucosal surfaces of the body of vitamin A deficiency includes the bulbar conjunctiva. In chronic deficiency, xerosis of the conjunctiva appears as a dry, nonwettable, rough or granular surface, best seen on oblique illumination from a hand-light. It is an unreliable sign because of observer variability and possible environmental causes. On the ocular surface, the tear film breaks up, revealing a xerotic surface. Histologically, the lesions represent a transformation of normal surface columnar epithelium, with abundant mucus-secreting goblet cells, to a stratified squamous epithelium that lacks goblet cells.
In advanced xerosis, grey to yellowish white foamy patches of keratinized cells and saprophytic bacilli, called Bitot’s spots, may aggregate on the surface, usually on the temporal conjunctival surface and, in more severe cases, on nasal surfaces as well. Bitot’s spots appearing in preschool children generally respond to high-potency vitamin A within 2–5 days and disappear within 2 weeks, although they may persist as smaller aggregates for months and, in some cases, X1B may be refractory to vitamin A and may reflect past rather than present history.
Corneal xerosis, ulceration and necrosis
Corneal xerophthalmia represents an acute decompensation of the corneal epithelium and is a sight-threatening medical emergency. Corneal xerosis quickly responds to vitamin A, usually within 2–5 days of therapy, with the cornea returning to normal without permanent sequellae within 1–2 weeks. Untreated corneal xerosis leads to blindness in one eye or both eyes. Shallow, small corneal ulcers, usually peripheral to the visual axis, can heal with minimal structural damage or risk of visual loss. Ulcers will heal to form an opaque scar (leukoma). Ulcers that perforate are plugged with iris and will, on healing, form an adherent leukoma; as these are often at the periphery of the cornea, central vision of the healed eye is left intact. Corneal necrosis (keratomalacia) must be treated immediately with standard vitamin A therapy, coupled with topical antibiotics and other nutritional measures (see Section 11.4, Treatment) or will result in meltdown of the eye with irreversible blindness in that eye.
Immunocompetence
Effects on the immune system
Not only does vitamin A deficiency reduce the barrier function of mucous membranes, there is also a significantly impaired systemic immune response in marginally deficient children. This improves markedly following vitamin A supplementation. Vitamin A deficiency has been described as a nutritionally acquired immunodeficiency disorder, one characterized by widespread pathological alterations of the mucosal epithelia of the respiratory, gastrointestinal and genitourinary tracts, impaired antibody responses to protein antigens, altered cell-mediated immunity and alterations in T-cell subsets. Vitamin A, through its active metabolite, all-trans-retinoic acid, regulates genes through nuclear receptors in the steroid and thyroid hormone superfamily. The genes relevant to immunity that are regulated by vitamin A have not been completely characterized, but probably include those of interleukin-4, interferon-γ, interleukin-2 receptor and others. Growth and activation of T- and B-cells are dependent on vitamin A, and vitamin A supplementation is associated with increases in circulating lymphocytes, including CD4 T-cells and natural killer cells, suggesting that lymphopoiesis is enhanced by vitamin A.
Infection
Vitamin A deficiency predisposes individuals to severe infection and, indeed, vitamin A has long been known as an anti-infective vitamin, given the evidence of numerous synergistic effects with infection on the host. Preschool children with mild xerophthalmia have a two to three-fold higher risk of respiratory infection and diarrhea than their nonxerophthalmic peers. In a prospective study in Indonesia, preschoolers with either diarrhea or acute respiratory disease were twice as likely to have developed xerophthalmia in a subsequent 3 month period than healthier children. Severe diarrhea, dysentery, measles and other severe, febrile illnesses are frequently reported to precede corneal xerophthalmia. Vitamin A deficiency and infection interact within a vicious cycle, whereby one exacerbates and increases the vulnerability to the other.
Infection can induce vitamin A deficiency through a variety of ways, depending on the cause, duration and severity of infection, and the vitamin A status of the host at onset. Serum retinol may be depressed following infection because of decreased dietary intake or absorption due to diarrhea or intestinal pathogens, impaired or accelerated hepatic depletion of retinol reserves, increased retinol utilization by target tissues or increased urinary losses associated with the acute-phase response. Urinary retinol loss, reflecting losses in body stores, depends on the type and severity of infection. In a study in Bangladesh, 6% of children hospitalized with dysentery and 65% with sepsis excreted approximately 10% of a preschool child’s estimated metabolic requirement. The interaction of vitamin A deficiency with diarrhea, measles and respiratory disease is well established, and it is increasingly likely with human immunodeficiency virus (HIV) infection and malaria, and to be associated with infectious episodes surrounding pregnancy, birth and the postnatal period.
Morbidity
Early work in Indonesia, particularly by Oomen and colleagues, recognized the high levels of vitamin A deficiency in children in the developing world and its impact on childhood blindness. Community-based observations by Sommer and colleagues in the early 1980s revealed that Indonesian children with mild xerophthalmia (XN or X1B), with no other obvious nutritional stress, were two to three times more likely to develop diarrhea or respiratory infection than those without xerophthalmia. They were also more likely, in a dose–response fashion in relation to eye signs, to die over the ensuing 4 months than children without any xerophthalmic eye signs. The epidemiology of the condition is discussed in greater detail below, but other factors involved in those at risk of vitamin A deficiency and infection, often as indicated by xerophthalmia, include age, gender, and socioeconomic and environmental factors.
In subclinical vitamin A deficiency, the incidence and prevalence of diarrhea may also increase. Mortality in children who are blind from keratomalacia or who have corneal disease is reported to be 50–90%, and measles mortality associated with vitamin A deficiency is increased to 50%. Several meta-analyses provide convincing evidence that community-based improvement in the vitamin A status of deficient children aged 6 months to 6 years reduces their risk of dying by 20–30% on average.
Amidst the consistent overall effects on child mortality, two seemingly incongruent observations exist. First, in contrast to evidence relating vitamin A deficiency to respiratory tract compromise and infection, vitamin A supplementation has not had a consistent effect in reducing the incidence, severity or mortality of acute lower respiratory infection in children. Secondly, vitamin A supplementation of infants under 6 months of age, provided either directly or indirectly through maternal provision, has not been shown to reduce mortality in early infancy (although one study in Indonesian neonates did show a 64% reduction in infant mortality). This apparent lack of effect concurs with a similarly observed lack of effect on early infant diarrheal and respiratory morbidity. Although shown to be safe (at 25 000 IU with immunization contacts in the first 6 months of life), the inconsistency in the effect of vitamin A supplementation on survival in early infancy, in populations at varying risk, has not been resolved. It may be that the amount of vitamin A given has been inadequate, or that other factors are involved. In Section 11.4, it will be seen that a consensus is emerging that, at the very least, it is necessary to ensure that the infant has adequate stores for the second 6 months of his or her life.
Children with severe measles will benefit from vitamin A therapy. Cases should be provided with the same treatment regimen as for children with xerophthalmia, according to the WHO United Nations Children’s Fund (UNICEF)/International Vitamin A Consultative Group (IVACG) (1997) guidelines. These have recently been updated (see Section 11.4). Such treatment will, under most conditions, reduce the severity of complications, accelerate recovery and lower case fatality.
A strong dose–risk gradient exists between maternal serum retinol and vertical transmission of HIV and cervicovaginal shedding of HIV DNA, suggesting that maternal vitamin A deficiency may affect pregnancy outcomes in HIV-positive populations. Unfortunately, vitamin A supplementation of HIV-positive pregnant women has shown little effect on outcomes such as low birth weight or perinatal mortality, or in interrupting the transmission of HIV from mother to infant. It seems very possible that low vitamin A levels (and possibly other micronutrients such as zinc) in pregnant women lead to a greater risk of puerperal sepsis.
Growth
Experimental vitamin A depletion in animals causes an initial deceleration in weight gain, which then plateaus as hepatic retinol reserves become exhausted, and eventually loss of weight. It is less clearly seen in children. Corneal xerophthalmia is associated with severe linear growth stunting and acute wasting malnutrition. Mild xerophthalmia (XN or X1B) is associated with moderate stunting and mild wasting, although associated comorbidity and nutritional deficiencies also restrict growth.
Field trials have shown mixed effects of vitamin A on child growth. Several have failed to find an overall effect of vitamin A supplementation on weight or height gain. Others have observed increments in linear, but not ponderal growth, or subgroup increments in both aspects of growth. Additional studies and stratified analyses suggest, however, that vitamin A can improve growth in children for whom vitamin A deficiency is likely to be growth limiting. Seasonal variation can affect the growth response to vitamin A supplementation.
Associations with protein–energy malnutrition and other micronutrient deficiencies
Protein–energy malnutrition
As protein adequacy is a prerequisite for optimal transport and utilization of vitamin A, serum retinol levels are depressed in the presence of wasting protein–energy malnutrition (PEM). Signs of vitamin A deficiency can also occur as a secondary phenomenon to PEM, regardless of whether or not the intake of vitamin A is adequate. This is due to impairment of the synthesis of plasma RBP which is then not available to transport retinol. This contributes to the severely impaired immune response to infection seen, as a result of both the functional vitamin A deficiency and the added impairment of immune responses associated with undernutrition.
Other micronutrients
Several other micronutrients have interactions with vitamin A of public health significance, most notably iron, but also zinc and selenium, and there may be some linked pathway with iodine. A lack of vitamin A can affect iron metabolism when deficiencies of both nutrients coexist and particularly when there is a high infectious disease load. The maximum hemoglobin response occurs when iron and vitamin A deficiencies are corrected together. Vitamin A deficiency appears to influence the availability of storage iron for use by hematopoietic tissue.
Mortality
Infants and children
Since 1986, eight population-based vitamin A-child mortality intervention trials, enrolling more than 165 000 children, have been conducted in south-east and south Asia and Africa (Figure 11.2). Results of metaanalyses based on these trials show that, in areas of endemic vitamin A deficiency, mortality of children 6–71 months of age can be reduced, on average, by 23–34% following vitamin A supplementation. This important effect can be partly explained by an ability of vitamin A to lower case fatality from measles by approximately 50%, as observed in field trials and hospital-based measles trials, mortality from severe diarrhea and dysentery by around 40% and, based on morbidity findings from a recent supplementation trial, possibly falciparum malaria. Combining mortality effects with data on the prevalence of vitamin A deficiency, it is estimated that 1.3–2.5 million early childhood deaths each year can be attributed to underlying vitamin A deficiency.
Women of reproductive age
The influence of vitamin A on survival has recently been extended to the reproductive years of women. An estimated 10–20% of women living in rural, malnourished populations of south Asia experience night blindness during pregnancy or lactation. Beyond being symptomatic of vitamin A deficiency, its occurrence during pregnancy appears to reflect a state of chronic vitamin A deficiency, anemia and wasting undernutrition, and an increased risk of infection and reproductive morbidity, and is associated with lower maternal survival during the first 1–2 years following delivery. Thus, in malnourished populations of women of childbearing age, improved vitamin A intake, through supplementation, and presumably by dietary means, may substantially reduce the risk of mortality related to pregnancy.
Figure 11.2 Results of eight population-based trials showing vitamin A mortality reduction after supplementation with vitamin A. Adapted from West and Darnton-Hill (2001).
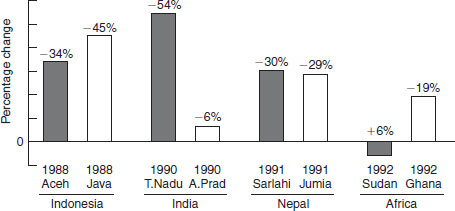
A study in Nepal, where night blindness is prevalent in pregnant women, pregnant and lactating women who were given 7000 μg RE (~23 300 IU) weekly demonstrated a decrease in prevalence of night blindness and a decrease in maternal mortality. The study is currently being replicated in several centers in Afica and Asia. This recent evidence of relatively high prevalences of vitamin A deficiency in women in the developing world, and the health impact of this situation, has encouraged many in international public health to examine new paradigms in the prevention and control of vitamin A deficiency.
11.3 Epidemiology
Magnitude of the problem
Vitamin A deficiency is, after PEM and iron-deficiency anemia, the most widespread and serious nutritional disease among young children. In the early 1990s, the WHO estimated that globally nearly 14 million children were affected annually by xerophthalmia and 190 million were at risk of subclinical vitamin A deficiency. This estimation was based largely on the appearance of clinical eye signs from which an extrapolation was made to estimate the total at-risk population. In 1994, the WHO updated the information on the magnitude of the vitamin A deficiency problem based on biochemical evidence of subclinical vitamin A deficiency (population-based blood levels of vitamin A ≤ 0.70 μmol/l), supported by other biological indicators and ecological risk factors such as poor diet. The 1994 global estimate indicated that 2.8 million preschool children are clinically affected by vitamin A deficiency and 251 million more are subclinically deficient. Thus, a possible 254 million preschool children are at risk in terms of their health and survival. The global distribution of vitamin A deficiency is shown in Figure 11.1, based on the number of preschool children at risk of or suffering from vitamin A deficiency.
Two regions, Asia and Africa, account for nearly 90% of the global problem. Although the prevalence of severe clinical vitamin A deficiency declines with age, less severe forms of vitamin A deficiency are also found in adolescence and young adults and women of reproductive age, where the prevalence of mild xerophthalmia may exceed the rates in preschool children. It is important to note that the estimate of the magnitude of the problem did not include adolescents or women of reproductive age, and it is likely that the size of the problem has been underestimated in this way (although a subsequent estimation has lowered the figure for the number of young children at risk to approximately 140 million).
Risk factors
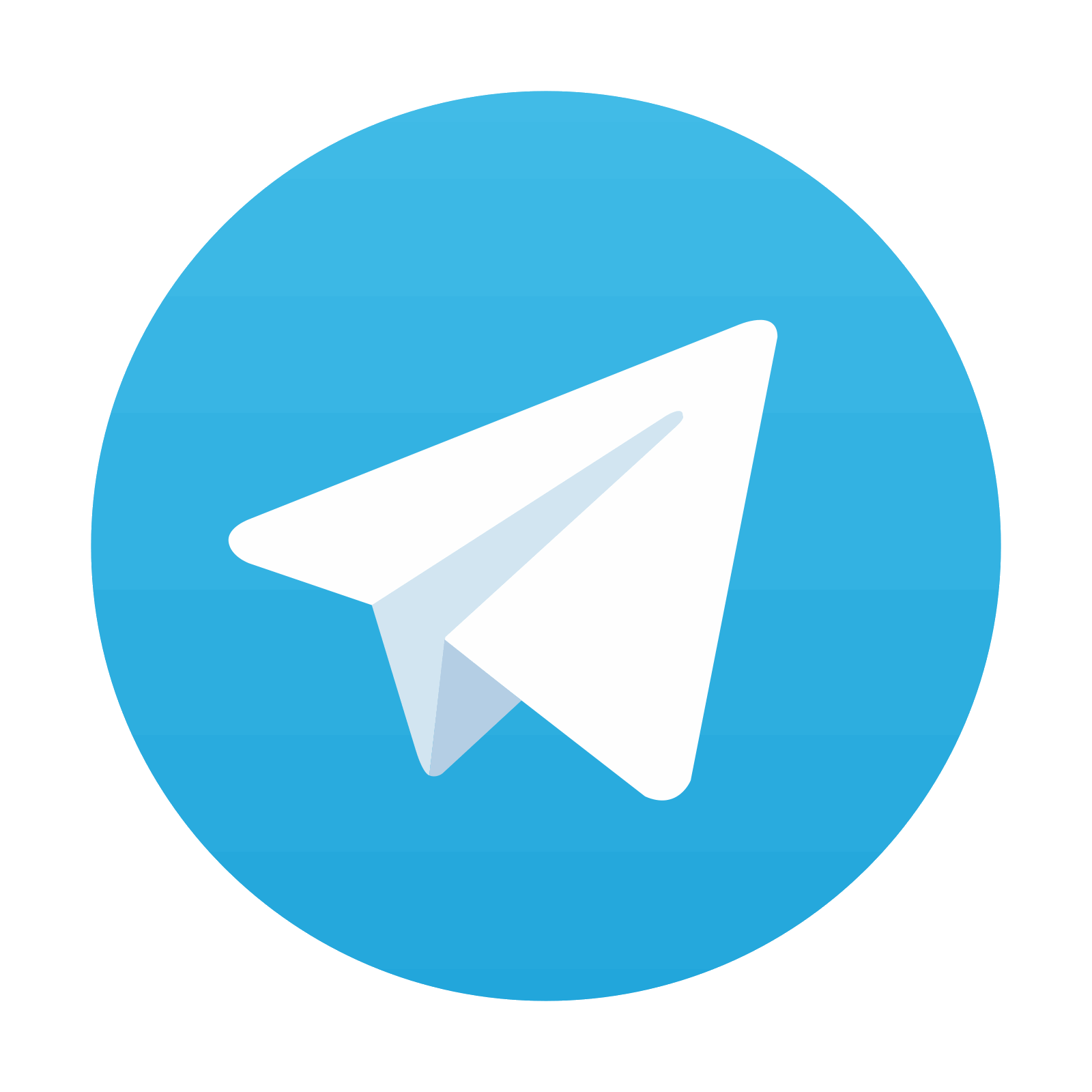
Stay updated, free articles. Join our Telegram channel
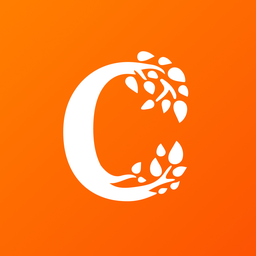
Full access? Get Clinical Tree
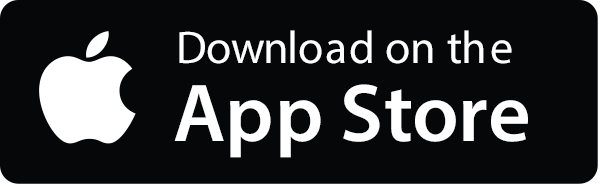
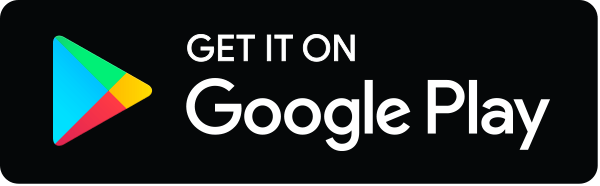