Vibration and Acoustics
Suzanne D. Smith
Jerry R. Goodman
Ferdinand W. Grosveld
The authors dedicate this chapter to the late Dr. Henning von Gierke
Aerospace systems generate some of the most severe vibration and noise environments encountered by humans. These dynamic environments, either singly or in combination, threaten the comfort, performance, and psychological well-being of persons associated with or exposed to aerospace operations. In more severe and/or prolonged periods of exposure, these environments become a health or occupational hazard, causing serious degradations in performance, modifications of physiological functions, and injury, including chronic back pain and hearing loss. Although relatively simple and straightforward methods are used to control moderate vibration and noise, the development of more complex control and mitigation methods and procedures requires a more in-depth understanding of the mechanisms involved in the generation of undesirable symptoms. Human vibration and bioacoustic research have directed attention to delineating the psychological, physiological, and performance effects of vibration and noise exposures on humans. The extensive knowledge and databases gathered from these studies serve as the basis for exposure guidelines and standards and for the development of predictive tools for estimating the environmental effects. Technological advances in vehicle design and human-integrated equipment continue to challenge researchers, designers, and health experts in ensuring effective aircrew performance, communication, and occupant safety during vibration and noise exposures. A significant portion of this chapter is reflective of the basic tools and knowledge associated with human response to vibration and noise presented in previous editions that have not dramatically changed over time. This edition expands on the issues and problems relevant to current and future aerospace systems.
WHOLE-BODY VIBRATION IN AEROSPACE ENVIRONMENTS
General Vibration Terminology
Vibration
Vibration is oscillatory motion in dynamic systems. A dynamic system possesses mass and the capability for relative motion between parts of the system, having the property of elasticity. Oscillatory motion can include periodic motion, in which the motion repeats itself in a given time period, or aperiodic, in which the motion does not repeat itself. In its simplest form, periodic vibration can be described as sinusoidal motion. Aperiodic vibration includes shock or transient motions. Random vibration is a type of motion described by its statistical properties that never exactly repeats itself. Stationary random vibration is characterized by statistical properties that can be estimated to be time-invariant. Nonstationary random vibration changes with time and is unpredictable. Most vibration encountered in aerospace environments is aperiodic or random.
The dynamic system of concern is the human body. In aerospace operations, oscillatory motion in the human body can be generated through structure-borne vibration or airborne vibration. Structure-borne vibration results from contact of the body with a physical structure such as a vehicle floor, seating system, or other equipment. Airborne vibration results from contact of the body with sound pressure waves produced during the airborne transmission of acoustic energy (also referred to as vibroacoustics).
Frequency
For simple periodic or sinusoidal vibration, the frequency of the motion is defined as the number of complete cycles of motion occurring in a unit of time, usually 1 second. The reciprocal of the frequency is the period of the motion, or the time associated with completing one cycle. The international unit for frequency is the Hertz (Hz), which is one cycle per second. Random vibration is also described in terms of its frequency content or frequency spectra by using appropriate spectral analysis techniques.
Amplitude
Amplitude or intensity is the measure of the system oscillatory motion about a position of rest. The amplitude of vibration can be described as displacement, velocity, or acceleration. For translational vibration, displacement is expressed in the unit meter (m). Velocity is expressed in units of meters/second (m/s). Acceleration is expressed in units of meters per second squared (m/s2). For rotational vibration, displacement is expressed in units of radians (or degrees). Velocity is expressed in terms of radians per second and acceleration is expressed in terms of radians per second squared. For simple sinusoidal motion, the relationships among displacement, velocity, and acceleration are:


where ω is the angular frequency in radians per second, f is the frequency in Hz (ω = 2πf), and x(t) is the instantaneous displacement at time, t. The amplitude of a sinusoidal motion can be expressed as a peak or peak-to-peak value. However, with random vibration, a time-averaged or root-mean-square (rms) value is commonly used. The rms value is calculated as
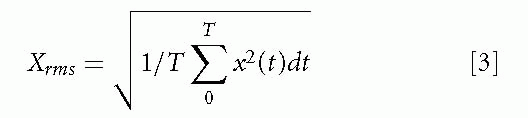
where x is the amplitude at time t, and T is the period of the vibration or length of time over which the rms value is being determined. The rms value of a sinusoidal motion is conveniently calculated as 1/√2 or 0.707 times the peak value. Acceleration is the most common unit used to describe vibration or oscillatory motion, particularly with regard to human exposure, due to the widespread and convenient use of acceleration transducers for measuring the motion. Acceleration is sometimes expressed in terms of g or the acceleration resulting from gravity. One g is equal to 9.80665 m/s2 depending upon the location on earth.
Resonance
When a sinusoidal excitation force is applied to a simple mass-spring-damper system (one degree-of-freedom), the system will initially vibrate at its natural frequency (free vibration), as well as at the frequency of excitation. When damping is present, the motion associated with the natural frequency will die out (transient motion) but motion at the excitation frequency will continue (steady state) as long as the force is present. If the excitation force is equal to the natural frequency of the system, resonance occurs. Without damping, the oscillations continue to build up, usually with catastrophic consequences. With damping, the oscillations will have finite amplitude. Resonance also occurs during exposure to random vibration that includes the natural or resonance frequency of the system. When the dynamic system is complex and described as a multi-degree-of-freedom system, each subsystem associated with a degree-of-freedom has the potential for excessive oscillations at its resonance frequency.
Direction
A body or system can move in translation, rotation, or a combination of both. Translational vibration occurs when a body or system oscillates along a straight line or in a linear direction (sometimes referred to as rectilinear motion.) The translational vibration of a body or system is defined along three perpendicular lines or orthogonal axes and include the fore-and-aft (X), lateral (Y), and vertical or longitudinal (Z) directions. Rotational vibration occurs when a body or system oscillates or rotates about a linear axis. Roll occurs when the body or system rotates about the X-axis, pitch occurs when the vibration is about the Y-axis, and yaw occurs when the rotation is about the Z-axis. The directions from which vibration enters the human body have been standardized by the International Standardization Organization [ISO 2631-1: 1997(E)] (1) and are illustrated in Figure 5-1. The human body is sensitive to the direction of vibration. Therefore, the perpendicular or orthogonal axes of vibration move with the body for movement from a seated or standing orientation to a recumbent orientation. The human body can translate and rotate either in or about a single axis or in a combination of up to six axes (X, Y, Z, roll, pitch, and yaw).
Spectrum
The spectrum of vibration represents the distribution of amplitude across frequency. This distribution is described in frequency bands that may be proportional or of constant bandwidth. The one-third octave bandwidth is the proportional bandwidth typically used when assessing human vibration exposure. Examples of constant bandwidths include spectra defined in increments of 0.1 Hz, 0.25 Hz, and
0.5 Hz. The most common unit used to describe the spectral components associated with human vibration exposure is the root-mean-square acceleration in m/s2 rms.
0.5 Hz. The most common unit used to describe the spectral components associated with human vibration exposure is the root-mean-square acceleration in m/s2 rms.
![]() FIGURE 5-1 Basi-centric coordinate system of the human body. International Standards Organization (ISO). Mechanical vibration and shock—evaluation of human exposure to whole-body vibration—part 1: general requirements. ISO 2631-1:1997(E), 1997. [© ISO. This material is reproduced from ISO 2631-1 with permission of the American National Standards Institute (ANSI) on behalf of the International Organization for Standardization (ISO). No part of this material may be copied or reproduced in any form, electronic retrieval system or otherwise or made available on the Internet, a public network, by satellite or otherwise without the prior written consent of the ANSI. Copies of this standard may be purchased from the ANSI, 25 West 43rd Street, New York, NY 10036, (212) 642-4900, http://webstore.ansi.org.] |
Duration
In general, human tolerance to continuous vibration declines with increasing duration of exposure. Although the timedependent effects of vibration are still not fully understood, it is generally accepted that long exposures at higher vibration levels may present a health risk, particularly when the exposures occur repeatedly over long periods. The timedependent effects form the basis for exposure criteria and standards as described later in this chapter.
Aerospace Sources of Whole-Body Vibration
Air Vehicle Propulsion System
The primary internal source of vibration in aerospace vehicles is the propulsion system. In conventional propeller-driven aircraft, vibration between 10 and 1,000 Hz is generated through the unbalanced forces associated with the engine speed and the propeller blade passage frequency. Blade passage frequency is calculated as the product of the propeller rotation speed (or propeller rotation frequency) and number of blades. In rotary-wing aircraft (rotorcraft) such as helicopters, propeller rotation speeds (or rotor speeds) range primarily between 200 and 400 revolutions per minute (rpm) or approximately 3 to 7 Hz. The numbers of blades typically range from two to four, producing blade passage frequencies mainly between 6 and 28 Hz, although higher blade numbers do exist. Blade imbalances can cause vibration at frequencies that are multiples of the propeller rotation speed, that is, 2 per revolution (2P), 3 per revolution (3P), and so on. Vibration can also be generated as multiples or harmonics of the blade passage frequency. Other propeller-driven aircraft have higher propeller rotation speeds and higher blade passage frequencies. For example, the six-bladed C-130J aircraft has a propeller rotation speed (or frequency) of 17 Hz. The blade-passage frequency is 102 Hz (6 × 17). Figure 5-2 illustrates the frequency spectra measured beneath the seat of a crewmember onboard a two-engine, four-bladed military propeller aircraft. The data were analyzed in 0.5 Hz increments. In this case, the propeller rotation speed (or
frequency) was approximately 18.5 Hz, and the blade passage frequency was approximately 73.5 to 74 Hz. The structure-borne vibration generated at these frequencies is transmitted to the occupants through a seating system or other surface in contact with the body, can be felt by the occupant, and persists to varying degrees throughout the flight. Jet engines operate at higher speeds, minimizing the potential for generating low frequency, structure-borne vibration. In space vehicles, the primary internal source of vibration is combustion in the large, multistage rockets used to launch the vehicle.
frequency) was approximately 18.5 Hz, and the blade passage frequency was approximately 73.5 to 74 Hz. The structure-borne vibration generated at these frequencies is transmitted to the occupants through a seating system or other surface in contact with the body, can be felt by the occupant, and persists to varying degrees throughout the flight. Jet engines operate at higher speeds, minimizing the potential for generating low frequency, structure-borne vibration. In space vehicles, the primary internal source of vibration is combustion in the large, multistage rockets used to launch the vehicle.
During aircraft engine run-ups and ground-based maneuvering, ground operations and maintenance crews can be exposed to airborne vibration that is transmitted to the body through acoustic waves. The noise levels associated with airborne vibration can be substantial in military environments where ground crews are required to work close to powerful aircraft and in restrictive areas. Such a hostile vibration environment is also found on aircraft carriers.
Air Turbulence
Air turbulence is the major external source of structure-borne vibration in aerospace vehicles. Weather and thermal effects contribute to air turbulence during flights up to 10,000 m (32,808 ft). Wind shear occurring between moving air masses can cause clear-air turbulence at altitudes above 10,000 m. Ground turbulence, influenced by the local heating and cooling between the air and ground surface and by wind effects, is prevalent at altitudes below 500 m (1,640 ft). Ground turbulence is particularly important during tactical operations. Such operations can require the pilot to fly at low altitudes (below 500 m) at high speeds. Helicopters are affected by ground turbulence because they typically operate near the ground surface. In addition, vibration in these aircraft can also occur because of ground effects caused by the coupling between the downwash and rotating blades (2).
Air Vehicle Structural Resonance
Buffeting is the vibration of a vehicle as it interacts with the air in which it moves, generating aerodynamic forces on the structure. These forces can excite the various modes of vibration or resonances of the vehicle (including fuselage and wing bending). It is not uncommon to encounter buffeting during commercial jet aircraft flight because of bad weather, thermal disturbances, or clear air turbulence. Although occurring rarely, such vibration can be severe enough to affect control of the aircraft. Buffeting also occurs in military high performance jets during low-altitude, high-speed flight, and aerial combat maneuvers (ACMs). This low-frequency buffeting can be severe but short in duration. It can act as a control cue to the pilot during these maneuvers. However, the transmission of buffet vibration to the aircrew may cause involuntary motions in the body and affect the operation of critical equipment (3).
Space vehicles are affected by aerodynamic forces, particularly during the first few minutes of acceleration during launch. During launch, structural vibration can occur primarily between 2 and 15 Hz due to the excitation of lateral bending and longitudinal oscillatory motions (2). During reentry, the space vehicle is decelerated by atmospheric friction. Given modern day control systems, reentry into the earth’s atmosphere is relatively smooth, although flight instabilities could cause relatively severe but short-term structural vibration (2).
Measurement and Analysis Techniques
Vibration Measurement Equipment
There are three components required to measure vibration: a transducer, signal conditioner or amplifier, and recording device. The transducer is an acceleration, velocity, or displacement detector that can measure the vibration in the various axes of translation and/or rotation. Accelerometers are the most commonly used transducers for measuring vibration because of their small size and light weight. Miniature accelerometers have been mounted onto major body anatomic structures such as the head. A semirigid disk with embedded accelerometers is commonly used to measure the vibration at the interface between the human body and contact surface, particularly in the seated position. The signal conditioner or amplifier is used to amplify the analog signal. Typically, the amplifier will produce an output in volts per unit of acceleration (when using an accelerometer). The signal conditioner may also be capable of filtering the analog
signal to attenuate certain low frequencies (high pass filter) or high frequencies (low pass filter). Vibration signals are commonly converted to digital signals and stored on a digital tape recorder, computer, or other digital storage media.
signal to attenuate certain low frequencies (high pass filter) or high frequencies (low pass filter). Vibration signals are commonly converted to digital signals and stored on a digital tape recorder, computer, or other digital storage media.
A variety of vibration meters is available for evaluating human vibration exposure. The features of this equipment usually conform to vibration standards requirements for data collection, filtering, and processing. The vibration is typically collected at the interface between the human and contact surface as described in the preceding text.
Vibration Analysis
Spectral Analysis Techniques
Human vibration is typically evaluated as a function of frequency because the human body is very sensitive to the frequency of motion. Analog frequency or spectrum analyzers use calibrated narrow band, octave band, or fractional-octave band filters to estimate the spectral content of an analog time history signal. Digital spectral analysis uses the fast Fourier transform (FFT) to estimate the spectral content of a digital time history signal. The spectral density (also known as the power spectral density or PSD) is the mean square of the signal per unit frequency and is widely used to present and compare the frequency spectra of excited systems exposed to random vibration. When the measurement is acceleration, the units are (m/s2)2/Hz. The root-mean-square or rms value can be calculated by multiplying the mean square value by the width of the frequency band over which the measurement was integrated and taking the square root. Caution must be taken when applying the more common digital spectral analysis to estimate the spectral content. Digital signals are sampled at a defined sampling interval, Δ (seconds) or frequency, 1/Δ (Hz). The Nyquist or folding frequency [1/(2Δ) Hz] is defined as one-half the sampling frequency (1/Δ Hz) and is the highest frequency that can be detected using the sampling interval, Δ (seconds). In order to avoid distortion or aliasing in the spectrum, the sampling interval should be small enough so that 1/2Δ Hz is greater than the highest frequency component expected in the signal. When the highest frequency component in the measurement is unknown, antialiasing techniques are typically applied during processing of the data. There are several methods described in signal processing texts and computer software packages for estimating the power or autospectral density, as well as the cross-spectral density calculated between various measured responses of a system. It is cautioned that the application of analog or digital processing techniques assumes that the vibration can be approximated as stationary or time invariant.
Transfer Functions
The transfer function defines the vibration transmission characteristics of a physical system. The transfer function is used to characterize the input/output relations between the source of vibration and the excited system as well as the relationship between motions of coupled components composing the system. In this regard, it can be a useful tool for formulating and validating mathematical models of the system response and for developing mitigation techniques and processes. The simplest case for calculating any transfer function is with a single input and single output measured in the same direction. For random vibration, the transfer function can be calculated as the ratio between the cross spectral density of the output and input measurements and the auto spectral density of the input measurement. This method produces a transfer function that describes the linear relationship between the input and output. The coherence function is a calculated value between 0 and 1; the closer the value is to unity, the more linear the relationship between the input and output with less contributions from noise and other sources unrelated to the input vibration.
The single-input/single-output transfer function has also been used to estimate the transmission characteristics when there is vibration in more than one direction. Alternative analytic methods for estimating the multiaxis transfer matrix are described in textbooks on signal processing.
Two transfer function methods are typically used to describe the transmission characteristics and resonances of the human body. They are the driving-point mechanical impedance and transmissibility methods. Driving-point (mechanical) impedance is defined as the ratio between the measured transmitted force and the input velocity of a vibrating system occurring in the same direction and at the same location (usually at the interface where the vibration enters the body, i.e., seat or feet). The units for the driving-point impedance magnitude are Newton-seconds per meter (N-s/m). A related function, the apparent mass, is used by several investigators to reflect the biodynamic characteristics of the body. The apparent mass is the ratio between the transmitted force and the input acceleration.
Peaks in the driving-point impedance magnitude and the phase relations between the input and output provide information on the frequency range(s) in which maximum energy is transmitted to the human body (i.e., body resonances) and in which maximum physiological and psychological effects may occur. The driving-point impedance also provides quantitative information on the effects of certain conditions and equipment (posture, restraint, seats) and can be used as a first clue to the mechanical structure of the human body and how to describe that structure in mechanical and engineering terms. However, impedance is primarily affected by the response of major components of the system and those components located nearest to the driving point or measurement location. Transmissibility is the ratio between input and output measurements of the same units. The transmissibility method provides valuable information on the transmission pathways and has been historically calculated from the output acceleration measured at the head and the input acceleration measured at the point of contact with the vibrating surface (seat or feet). The transmission of vibration and impact through the body structure is of primary interest with respect to explaining undesirable effects such as trauma and performance degradation. Peaks in the magnitude of the
transmissibility, like impedance, are associated with body resonances. Measurements at multiple locations can be used to estimate the resonance frequencies of system components and can, for the human body, provide coupling information between connected anatomic structures or regions. This information, as with impedance, is quite useful for developing and validating robust human vibration models (particularly mass-spring-damper or lumped-parameter models). For the human body, special consideration must be given to the weight, location, and method of attaching the transducer because these factors could affect the measurements.
transmissibility, like impedance, are associated with body resonances. Measurements at multiple locations can be used to estimate the resonance frequencies of system components and can, for the human body, provide coupling information between connected anatomic structures or regions. This information, as with impedance, is quite useful for developing and validating robust human vibration models (particularly mass-spring-damper or lumped-parameter models). For the human body, special consideration must be given to the weight, location, and method of attaching the transducer because these factors could affect the measurements.
Vibration Exposure Metrics
The transfer functions mentioned in the preceding text are used to describe the biodynamic responses of the human body. Characteristics of these responses are described in the section on Vibration Effects on Humans. There are human vibration standards that call for the use of specific metrics for assessing human exposure relative to health, safety, and comfort. The most common metric is the frequency-weighted rms acceleration. The acceleration is measured in all three orthogonal axes at the interfaces where the body contacts the vibrating structure. For the seated person, the interfaces include the surface between the buttocks and seat pan, the back and seatback, and the feet and supporting surface. For the standing person, the interface includes the feet and supporting surface. For the recumbent person, the interfaces include the pelvis and the supporting surface, and the head and supporting surface. A pliable disk with embedded accelerometers is typically used for these measurements. The measured acceleration is weighted in the time or frequency domain. In the time domain, the calculation is similar to Equation 3, where Xrms is now the weighted acceleration, aw. Using the acceleration spectra (estimated using the spectral methods described previously), the weighted acceleration can be calculated as
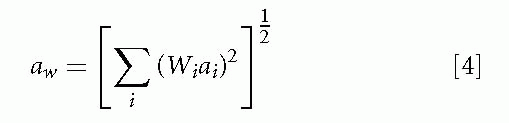
where Wi is the frequency weighting associated with the ith frequency band, and ai is the rms acceleration associated with the ith frequency band. The frequency weighting depends on the frequency, direction, and, in some cases, the location of the measurement. Historically, the frequency weighting curves were derived from equivalent comfort contours; the frequency weightings are high where the equivalent comfort contours are low (4). Researchers continue to evaluate human sensitivity to vibration. These efforts may result in a revision of the current frequency weightings. Additional manipulations of the weighted acceleration levels include the application of multiplying factors that reflect the relative effects of vibration direction, the use of the fourth-power instead of the second-power acceleration, and the vectorial summation of weighted acceleration levels for assessing vibration effects. Several of these metrics are described in the section on Exposure Guidelines and Regulations.
Vibration Effects on Humans
Human Body Biodynamics
The transfer function methods described earlier have been applied for characterizing human body biodynamics in vibration environments. Historically, most human whole-body vibration research and exposure assessments focused on measuring human responses during exposure to seated vertical vibration occurring along the vertical or longitudinal axis of the body (Z in Figure 5-1). The human body is most sensitive to vibration in the vertical direction. Some aerospace vehicles, such as propeller-driven aircraft and spacecraft, generate significant vibration in the horizontal directions. As mentioned in the previous section on vibration analysis, the two most common techniques used to evaluate the dynamic characteristics of the human body are the driving-point impedance (or apparent mass) and transmissibility.
Driving-Point Impedance/Apparent Mass
Figure 5-3 illustrates the driving-point impedance of one subject exposed to vertical vibration with several postures (5). At the low frequencies (below ˜3 Hz), the body acts like a pure mass corresponding to the body weight (represented theoretically by the line Z = mω). Depending on the distribution of body mass and the associated composition, muscle tension, and posture, there is a major peak in the impedance that usually occurs between 4 and 8 Hz. This peak is known as the primary whole-body resonance. It is specifically associated with relative motions in the upper torso and shoulder girdle, including the soft tissue and organs in the upper thoracoabdominal region. These relative motions cause the transmission of higher forces back to the measurement site at the seat-occupant (or floor-occupant) interface as compared with the response of a rigid mass (Figure 5-3). At higher frequencies above the primary resonance frequency, more energy is absorbed by the elasticities and damping inherent in the soft tissues. At the higher frequencies, the impedance tends to decrease, although other smaller regions of resonance can be identified, particularly around 10 Hz and 15 Hz as shown in Figure 5-3. These peaks have been, in general, associated with resonances of the spine but may be influenced by the response of other anatomic structures, including the legs. Figure 5-3 shows that the sitting erect posture results in the upward shift of the resonance frequency by approximately 1 Hz. Using this simple representation, it can be shown that the erect posture results in stiffening of the body. The impedance of the human body is linear only to a first approximation. For simultaneous exposures to both vibration and sustained acceleration (inertial preload) at +2 Gz and above, the primary body resonance shifts to higher frequencies between 8 and 10 Hz with a corresponding increase in the magnitude of the impedance peak (6). The changes observed in body biodynamics and resonance behavior may also affect human tolerance (see section on Physiology and Health). However, any strong vibration occurring in the 8 to 10 Hz range could have more serious consequences during G-preload because of the shift in body sensitivity under these conditions. In contrast to the effects
of sustained acceleration, studies have also shown that, under normal gravity, the primary resonance peak observed in the impedance shifts downward by 1 to 2 Hz with increases in the vibratory acceleration. The associated decrease in stiffness with higher vibratory acceleration is not as dramatic as the increase in stiffness associated with G-preload.
of sustained acceleration, studies have also shown that, under normal gravity, the primary resonance peak observed in the impedance shifts downward by 1 to 2 Hz with increases in the vibratory acceleration. The associated decrease in stiffness with higher vibratory acceleration is not as dramatic as the increase in stiffness associated with G-preload.
Transmissibility
The primary peak observed in the vertical seat-to-head transmissibility coincides closely with the primary resonance peak observed in the impedance data. The vertical head transmissibility decreases to relatively low values at higher frequencies above the whole-body resonance (when there is no direct contact with a vibrating surface). The transmissibility has also been calculated between the input at the seat and other body locations including the chest, spine, and legs (7). Caution should be taken in interpreting these results because the attachment of the transducer to the body surface may be affected by the response of the underlying muscle or skin. Accelerometers have become very lightweight and can minimize some of these effects. These data, along with the driving-point impedance data, provide valuable information on the coupling behavior occurring between anatomic regions. For example, the resonance peak observed in the impedance between 8 and 10 Hz for some subjects coincided with a peak transmissibility response in the unsupported legs (7). Peaks in the spine transmissibility have been observed around 6 Hz, 10 to 12 Hz, and 12 to 20 Hz, coinciding with peak regions observed in the impedance for some subjects and suggesting significant coupling effects between anatomic structures or regions.
Exposure to vertical axis vibration can result in other motions in the body depending on posture and head orientation. Head pitching in the forward-facing position is quite prevalent and is also observed during exposure to fore-and-aft vibration. Off-axis head orientations (other than the forward-facing position) can increase the transmission of vibration to the head. Head vibration can have significant effects on visual performance as discussed later in this chapter. In addition, resonance of the eye has been reported to occur between 20 and 70 Hz (4) and can contribute to visual degradation.
There have been an increasing number of women entering the predominantly male aerospace workforce (see Chapter 22). Because most subjects participating in research have been men, there is the need to understand similarities and differences in female/male responses to aerospace vibration for insuring optimum performance and safety of qualified individuals. Recent research has suggested that some differences may exist in the vibration response characteristics between women and men, but these differences have so far not resulted in definitive effects on performance, health, and safety relative to aerospace operations. The limited data have suggested that, in general, the distribution of the mass, stiffness, and damping characteristics of the major dynamic anatomic regions differs between women and men, but not to the extent of dramatically affecting the primary resonance frequency associated with upper torso motion (7).
Multiaxis Vibration
Although vertical vibration has been the major concern in aerospace operations, the presence of vibration in more than one direction can contribute to even greater and more complicated motions of the body. Research efforts to determine the effects of multiaxis or combined-axis vibration in aerospace operations are becoming more common with the availability of safe but sophisticated multiaxis vibration platforms and improved methods for quantifying occupant vibration during operations. As mentioned previously, there are analytic methods available for estimating the multiaxis transfer matrix when substantial vibration occurs in more than one axis.
Airborne Vibration
The mismatch between the acoustic impedance of air and the human body surfaces prevents significant amounts of acoustic energy from entering the body, particularly at higher frequencies (8). With decreasing frequencies below 1,000 Hz, more acoustic energy is absorbed in the form of transverse shear waves. With exposure to high-intensity noise levels (120 dB) between 100 and 1,000 Hz, tissue vibration occurs and the noise is felt through the stimulation of somatic mechanoreceptors (2). Below 100 Hz, intense noise can cause whole-body vibration that not only affects motion in the chest, abdominal wall, viscera, limbs, and head but can also generate motions in the body cavities and air-filled or gas-filled spaces (2). Von Gierke reported that resonance of the chest wall and air-filled lungs occurs around 60 Hz (8).
Previous studies investigating the effects of airborne vibration have directed attention to the association between noise level and the subjective assessment of the exposure (9). Lightweight accelerometers have been attached to the body surface for estimating body accelerations while standing near jet aircraft during engine run-ups (10). Figure 5-4 shows peak acceleration in the upper torso between 60 and 100 Hz, particularly in the fore-and-aft (X) direction of the chest for one subject. This peak coincides with the upper torso resonance reported by von Gierke and Nixon (8). It also appears that, under the conditions tested, the peak increases with increasing noise level. It should be cautioned that these preliminary data do not address the effects of airborne vibration on individuals of varying weight and stature.
![]() FIGURE 5-4 Acceleration peak observed between 60 and 100 Hz measured in the fore-and-aft direction of the chest during ground engine run-up tests in selected aircraft at military power (MP) and afterburner (AB). (Smith SD. The effects of airborne vibration on human body vibration response. Aviat Space Environ Med 2002;73(1):36-45.) |
Physiology and Health
The mechanical stresses imposed on the body during vibration exposure can potentially lead to interference with bodily functions and tissue damage in practically all parts of the body, depending on the frequency range and exposure conditions. Most aerospace vibration exposures remain below injury levels. Acute exposures to whole-body vibration, primarily in the range of 1 to 20 Hz (11), of intensities voluntarily tolerated by human subjects up to the limit of severe discomfort or pain, have not resulted in demonstrable harm or injury. Continued exposures at the limits of tolerance are considered to have a high potential for producing bodily damage. Figure 5-5 illustrates the short time, 1-minute, and 3-minute tolerance limits reported by healthy adult male subjects exposed to vertical sinusoidal vibration (11). The figure shows the decrease in tolerance with longer exposure periods. Minimal tolerance occurs between 4 and 8 Hz, the frequency range associated with whole-body resonance. Figure 5-6 depicts the frequencies at which the most severe symptoms have been reported. Headache is often associated with exposure to frequencies above 10 Hz. Particularly for exposures to sustained fore-and-aft acceleration (Gx) with gx or gy vibration (space couch positions), motions are transmitted to the head directly from the headrest, which can lead to extremely uncomfortable and disturbing impacts to the head. During launch and reentry of spacecraft, the crew is oriented in the semisupine posture. This minimizes the influence of sustained acceleration by directing the force through the fore-and-aft or X direction of the body (Figure 5-1). This does couple the body more closely to the seating system. The vibration tolerance associated with this seat orientation is discussed in the section Human-Equipment Interfaces.
Most physiological effects in the 2 to 12 Hz frequency range are associated with the resonance of the thoracoabdominal viscera. Movement of the thoracoabdominal viscera
in both X- and Z-axis excitation is responsible for the interference of vibration with respiration. It causes the involuntary oscillation of a significant volume of air in and out of the lungs, leading to an increase in minute volume, alveolar ventilation, and oxygen consumption. In experimental exposures to gz vibrations, pCO2 decreased and clinical signs of hypocapnia were observed, suggesting hyperventilation (2). As shown in Figure 5-6, dyspnea results from short exposures to high-amplitude vibration. Changes in cardiovascular functions, including arterial blood pressure, cardiac index, heart rate, and oxygen consumption index, were shown to be dependent on the amplitude and the frequency of whole-body vibration. In general, the combined cardiopulmonary response to vibration in the 2 to 12 Hz range resembles the response to exercise. Although increased muscular effort of bracing against the vibration and psychological factors may account for some of the response, observance of the same general pattern in anesthetized animals speaks for the stimulation of various mechanoreceptors (12).
in both X- and Z-axis excitation is responsible for the interference of vibration with respiration. It causes the involuntary oscillation of a significant volume of air in and out of the lungs, leading to an increase in minute volume, alveolar ventilation, and oxygen consumption. In experimental exposures to gz vibrations, pCO2 decreased and clinical signs of hypocapnia were observed, suggesting hyperventilation (2). As shown in Figure 5-6, dyspnea results from short exposures to high-amplitude vibration. Changes in cardiovascular functions, including arterial blood pressure, cardiac index, heart rate, and oxygen consumption index, were shown to be dependent on the amplitude and the frequency of whole-body vibration. In general, the combined cardiopulmonary response to vibration in the 2 to 12 Hz range resembles the response to exercise. Although increased muscular effort of bracing against the vibration and psychological factors may account for some of the response, observance of the same general pattern in anesthetized animals speaks for the stimulation of various mechanoreceptors (12).
![]() FIGURE 5-6 Symptoms experienced for frequencies between 2 and 20 Hz at tolerance levels. cps, cycles per second or Hertz. (Magid EB, Coermann RR, Ziegenruecker GH. Human tolerance to whole body sinusoidal vibration. Aerospace Med 1960;31:915-924.) |
The most commonly reported chronic health symptoms in occupations that include prolonged whole-body vibration exposure are back pain and back disorders. However, these symptoms are also reported for other occupations as well. The spinal column is a major pathway for the transmission of vibration in the upper torso. Repeated exposures to vibration can affect the mechanical integrity of the musculoskeletal components, leading to injury. In aerospace operations, the highest incidence of back pain and back disorders is reported
for helicopter pilots. Body posture is an important factor to consider in the generation of these symptoms. Helicopter pilots in particular may adopt poor sitting postures to operate the aircraft and to improve visibility (13,14).
for helicopter pilots. Body posture is an important factor to consider in the generation of these symptoms. Helicopter pilots in particular may adopt poor sitting postures to operate the aircraft and to improve visibility (13,14).
The symptoms of subjects exposed to airborne vibration were studied by the U.S. Air Force in the 1960s (9). In summary, for exposures below 150 dB, the most common symptom reported was mild-to-moderate chest vibration. From 50 to 100 Hz, pure tone exposures reached levels above 150 dB. Voluntary tolerance was reached at 50 Hz (153 dB), 60Hz(154 dB), 73 Hz(150 dB), and 100 Hz(153 dB) based on the significance of symptoms reported by the subjects. Symptoms included headache (50 Hz); coughing, severe substernal pressure, choking respiration, salivation, pain on swallowing, hypopharyngeal discomfort, and giddiness (60 Hz and 73 Hz); and mild nausea, giddiness, subcostal discomfort, cutaneous flushing, and tingling (around 100 Hz) (9). In the more recent study (10), reports of increasing chest vibration coincided with the increase in the peak chest acceleration and the increase in the noise level. It has been suggested that prolonged exposures to high-pressure, low-frequency noise may have physiological and pathological consequences.
Performance
Manual Control and Head Tracking
Low-frequency aerospace vibration that occurs during turbulence or buffeting in jet aircraft, helicopter operations, and launch and reentry of space vehicles present a particular challenge to manual control and head tracking performance. The involuntary motions of the body and the extremities introduced by the vibration are imposed as disturbances on the required control of the human operator. The greater the vibration amplitude of the hand, foot, or head in comparison with the required control motion (through strong original excitation or through resonance reinforcement), the larger the undesirable interference.
Laboratory manual control experiments have shown that manual tracking errors increase in the 2 to 16 Hz frequency range at seat accelerations above approximately 0.05 gz rms. The maximum decrement is usually in the range of 4 Hz, which is in the vicinity of the main body resonance (4 to 8 Hz). Above approximately 0.25 gz rms, manual control can be seriously affected and worsen with time due to fatigue (2). For X-axis and Y-axis vibration, the largest decrements are at 1.5 to 2 Hz. Caution should be taken in generalizing the decrements because they depend too much on the specific details of the control task (e.g., position, velocity or force control, amplitude of required control motion) and the hand-arm or foot-leg support. Early studies suggested that high-intensity noise between 100 and 105 dBA combined with vibration produced less decrement in manual tracking performance than with vibration alone. However, when 110 dBA noise was combined with vibration, the tracking performance was more degraded than observed for either noise or vibration alone (15).
The advent of helmet-mounted equipment requires the head to track and target objects. Low-frequency vibration that causes oscillations of the head can increase tracking and targeting error. Off-axis head orientations can further increase head motions and have been associated with increased head tracking error below 10 Hz (16).
The severity of the vibration interference can be influenced to some extent by the operator’s control strategy. For example, under turbulent flight conditions, pilots often postpone manual activity during short bursts of high-amplitude vibrations and introduce corrective action as soon as the burst is over. Under sustained turbulence, very low frequencies can excite “pilot-induced oscillations,” which are caused by inappropriate control inputs. The pilot apparently has time to correct for the disturbance inputs but, owing to misinterpretations of kinesthetic cues or the response characteristics of the motor system, he or she does not compensate in an appropriate way at some frequencies and adds to aircraft instabilities.
Visual Performance
A complex relationship exists among all of the relevant parameters affecting visual performance including vibration frequency, amplitude and direction, viewing distance, illumination, contrast, the shape of the viewed object, and the occupant posture and restraint. Difficulties in reading instruments and performing visual searches occur when vibration introduce relative movement of the eye with respect to the observed object or target, even when the observed object (such as the instrument panel) is excited by the same structural vibration. The greatest decrement in visual performance occurs with vibration of the object or display alone, followed by vibration of the occupant alone. The least relative effect occurs with vibration of both the occupant and the object or display (4).
Compensatory eye movement is the physical response to vibration and affects visual performance. During head rotation, the vestibulo-ocular reflex causes the eye to move in the opposite direction of the motion, thereby stabilizing the line-of-sight (LOS) to a stationary object (compensatory eye movement). The compensatory eye movement has been shown to be effective up to 8 Hz with some studies showing effectiveness up to 20 Hz (4). Compensatory eye movement is the most effective during head rotations. Exposure to translational vibration at lower frequencies is expected to produce both translation and rotation of the head. At higher frequencies, the eye resonance described previously can result in blurred vision. Given the damping effect of the body at higher frequencies, this effect may occur only where high levels of vibration are present or when the head comes in direct contact with a vibrating surface.
The launch and reentry phase of spaceflight presents a unique problem for visual performance because the crew is oriented in the semisupine posture with both body and head closely coupled to the seat and restraint system. This special case is discussed further in the section Human-Equipment Interfaces.
Cognitive Performance
Early studies strongly suggested that the performance of simple cognitive tasks (pattern recognition and monitoring of dials and warning lights) were not affected by vibration. More demanding tasks have shown to be affected by vibration and combinations of vibration and noise. One study involved mental arithmetic and short-term memory during a 0.5 gz (peak) vibration at selected frequencies between approximately 5 Hz and 16 Hz. The results showed significantly slower performance as compared to the static condition (17). Regardless of these findings, it has also been suggested that vibration can increase the level of arousal, similar to observations in noise, depending on the exposure intensity, exposure time pattern, and the subject activity.
As with manual tracking performance, studies have also been conducted on the combined effects of vibration and noise on cognitive performance. An early study suggested that either 100 or 110 dBA noise combined with vibration produced more adverse effects on the performance of a mental arithmetic task than either stressor alone. In another study using a complex counting task, it was found that the effect of noise was reversed with vibration. While 100 dBA noise as compared to 65 dBA noise produced greater performance degradation, the 100 dBA noise combined with vibration as compared to the 65 dBA noise combined with vibration produced less degradation (15). These results were similar to the results for manual tracking performance using similar noise levels.
Human-Equipment Interfaces
In aerospace operations, the human body becomes coupled with any interfacing equipment, including seating systems and helmet systems. Although this coupling could provide the mechanisms for minimizing vibration effects, current use of this equipment in aerospace environments has been associated with comfort, health, and performance degradation, exacerbating effects already described previously. For example, most conventional seat cushions tend to increase the transmission of vertical vibration from the seat to the upper torso and head in the vicinity of whole-body resonance (4 to 8 Hz), but dampen these motions at higher frequencies as compared to sitting in a rigid structure. Passive suspension systems have been used to attenuate vehicle vibration near human resonance but typically increase the transmission of vibration to the body at around 2 Hz and below, producing relatively large displacements at these frequencies. Active suspension systems use a feedback mechanism to stabilize the seat motion relative to the vehicle motion. In military aircraft that include ejection seats, the seat cushions are relatively thin and stiff, to minimize any rebound of the body during ejection or ground impact. Consequently, they provide little damping or isolation from any vehicle vibration. Current suspension or isolation systems may add unwanted weight and compromise crashworthiness in these types of aircraft. As mentioned previously, vibration transmitted directly from the headrest to the head can affect visual performance, causing visual blurring most likely related to eye resonance.
Helmet systems used in military flight environments can affect head motions. Increasing the mass of the helmet and increasing the distance between the center of gravity (CG) of the head/helmet system and the head have been shown to increase head pitch in the forward-facing direction (18). Sophisticated helmet-mounted visual systems including night vision goggles (NVG), helmet-mounted displays (HMDs), and helmet-mounted targeting and display (HMT/D) interfaces present unique issues in performance. For example, the compensatory eye movement is rendered ineffective in stabilizing the LOS to a viewed object when using an HMD because of the movement of the projected object with the head (19). It has been shown that reading error is greater with the use of an HMD as compared with a panel-mounted display (4). Relative head/helmet motion or slippage could further reduce the effectiveness of these systems. As mentioned previously, degradation in head tracking performance has also been associated with vibration (3,16). The low-frequency buffeting associated with maneuvering of certain fighter aircraft can cause substantial involuntary motions of the head and may compromise the performance of head tracking and targeting systems. The extreme head/helmet orientations that can occur during these operations exacerbate this issue.
The vibration encountered during launch and reentry of spacecraft presents a special case for human tolerance and visual performance in the semisupine posture, where coupling between the occupant and seating system is critical. Studies were conducted back in the 1960s to evaluate the effects of the seat or couch, body restraint, and head restraint on subjective tolerance and visual performance. Figure 5-7 illustrates the mean level of acceleration tolerance at the tested frequency for the seat or couch configurations (20). The exposure duration depended on the frequency; the higher the frequency, the less time to reach a given acceleration level. The longest tolerance exposure took 250 seconds or approximately 4 minutes. There was no helmet or head restraint used with the contoured couch. The subjects maintained their head in the headrest. If head buffeting became intolerable, they could lift their head and continue until voluntary tolerance was reached. A helmet system with restraint in the X and Y directions was used with the adjustable couch. The helmet restraint release was designed into the system. Once the subject released the helmet restraint, the exposure was discontinued and the associated acceleration level considered the voluntary tolerance. Figure 5-7 shows that the adjustable couch produced higher tolerance in the X-axis below approximately 10 Hz but flattened at higher accelerations, low tolerance in the Y-axis above approximately 8 Hz, and similar tolerance as compared to the contoured couch in the Z-axis. In the X-axis, the main focus of complaints was in the thoracic area, particularly with the contoured couch. The subjects reported difficulty breathing with both configurations. Repeated impact of the sacrum was reported for the adjustable couch, although, in general, the body was more coupled to this couch as compared to the contoured couch. These symptoms were
also reported for the Y-axis, but not as frequently. Frictional rubbing and banging were reported for the contoured couch in the Y direction. In the Z-axis, there were more complaints about the head, including headache and sore neck muscles. Raising the head appeared to isolate the head from higher frequency vibration in the contoured couch, but may have contributed to fatigue. The helmeted head and good coupling to the adjustable seat limited complaints at low frequencies but reduced tolerance at higher frequencies, particularly in the horizontal directions (20).
also reported for the Y-axis, but not as frequently. Frictional rubbing and banging were reported for the contoured couch in the Y direction. In the Z-axis, there were more complaints about the head, including headache and sore neck muscles. Raising the head appeared to isolate the head from higher frequency vibration in the contoured couch, but may have contributed to fatigue. The helmeted head and good coupling to the adjustable seat limited complaints at low frequencies but reduced tolerance at higher frequencies, particularly in the horizontal directions (20).
![]() FIGURE 5-7 Mean level of human acceleration tolerance for two spacecraft couches. Tolerance is shown for the three orthogonal directions of the recumbent occupant (Figure 5-1) at selected frequencies. (Redrawn from data presented in Temple WE, Mandel MJ, Clarke NP, et al. Man’s short-time tolerance to sinusoidal vibration. AMRL-TR-65-96, Wright-Patterson AFB, Ohio: Aerospace Medical Research Laboratories, 1965.) |
Dial-reading performance was investigated at 6, 11, and 15 Hz using a Mercury helmet (21,22) and Apollo helmet (23) in the semisupine posture. The Mercury helmet was tested in the X (+1 Gx ± 1.1 gx) and Y (+1 Gx ± 0.9 gy) directions in the first study and only in the X direction in the second study. The Apollo helmet was tested in both the X and Y directions at levels coinciding with those used in (21). The first study (21) included the unrestrained and restrained helmeted head. The second study (22) included the restrained head and a piston-spring damper. The third study (23) tested the helmet with and without a liner. In general, all three studies showed that coupling the head to the seat reduced the reading error at frequencies below 10 Hz. This also coincided with the increase in tolerance when restraining the head (20). The results suggested that isolating the head at frequencies above 10 Hz might improve reading performance and improve tolerance (22). It is cautioned that the early tolerance and performance studies were conducted in well-controlled laboratory environments with physically qualified military members.
Vibration Protection and Mitigation
Exposure Guidelines and Regulations
National and International Standards
Given the physiological and psychological effects of vibration described previously, it is clear that there are no simple assessment procedures and exposure limits that are applicable to all environmental, human posture and restraint, and task performance conditions. However, based on laboratory and field experiences, whole-body vibration standards have been developed that provide boundaries and guidelines for assessing the effects of vibration. The primary national standard for structure-borne vibration is the American National Standards Institute (ANSI) Guide for the Evaluation of Human Exposure to Whole Body Vibration (ANSI S3.18-2001) (24). The primary international standard is the International Standards Organization’s (ISO) Mechanical Vibration and Shock: Evaluation of Human Exposure to Whole Body Vibration, Part 1: General Requirements (ISO 2631-1: 1997) (1). The 2001 ANSI standard is identical to the ISO 2631-1: 1997.
The ISO 2631-1: 1997 uses the frequency-weighted accelerations and multiplying factors described in the section
Vibration Analysis for assessing the effects of vibration. For assessing the effects of vibration on health, the ISO 2631-1: 1997 provides health guidance caution zones as shown in Figure 5-8 (based on Equation B.1). Below the lower boundary zone, health effects have not been clearly documented or observed. Within the upper and lower boundary zones, caution is indicated for potential health risks. Above the higher boundary zone, health risks are likely. The highest rms value of the frequency-weighted acceleration determined in any axis at the seat pan is compared to these zones. In addition to a caution zone based on the weighted rms acceleration (second power), a caution zone is also provided on the basis of weighted fourth power vibration dose method. The vibration dose value (VDV) represents a cumulative exposure and is more sensitive to peaks in the exposure. It is noted that the emphasis of these standards is on the health risk to the lumbar spine.
Vibration Analysis for assessing the effects of vibration. For assessing the effects of vibration on health, the ISO 2631-1: 1997 provides health guidance caution zones as shown in Figure 5-8 (based on Equation B.1). Below the lower boundary zone, health effects have not been clearly documented or observed. Within the upper and lower boundary zones, caution is indicated for potential health risks. Above the higher boundary zone, health risks are likely. The highest rms value of the frequency-weighted acceleration determined in any axis at the seat pan is compared to these zones. In addition to a caution zone based on the weighted rms acceleration (second power), a caution zone is also provided on the basis of weighted fourth power vibration dose method. The vibration dose value (VDV) represents a cumulative exposure and is more sensitive to peaks in the exposure. It is noted that the emphasis of these standards is on the health risk to the lumbar spine.
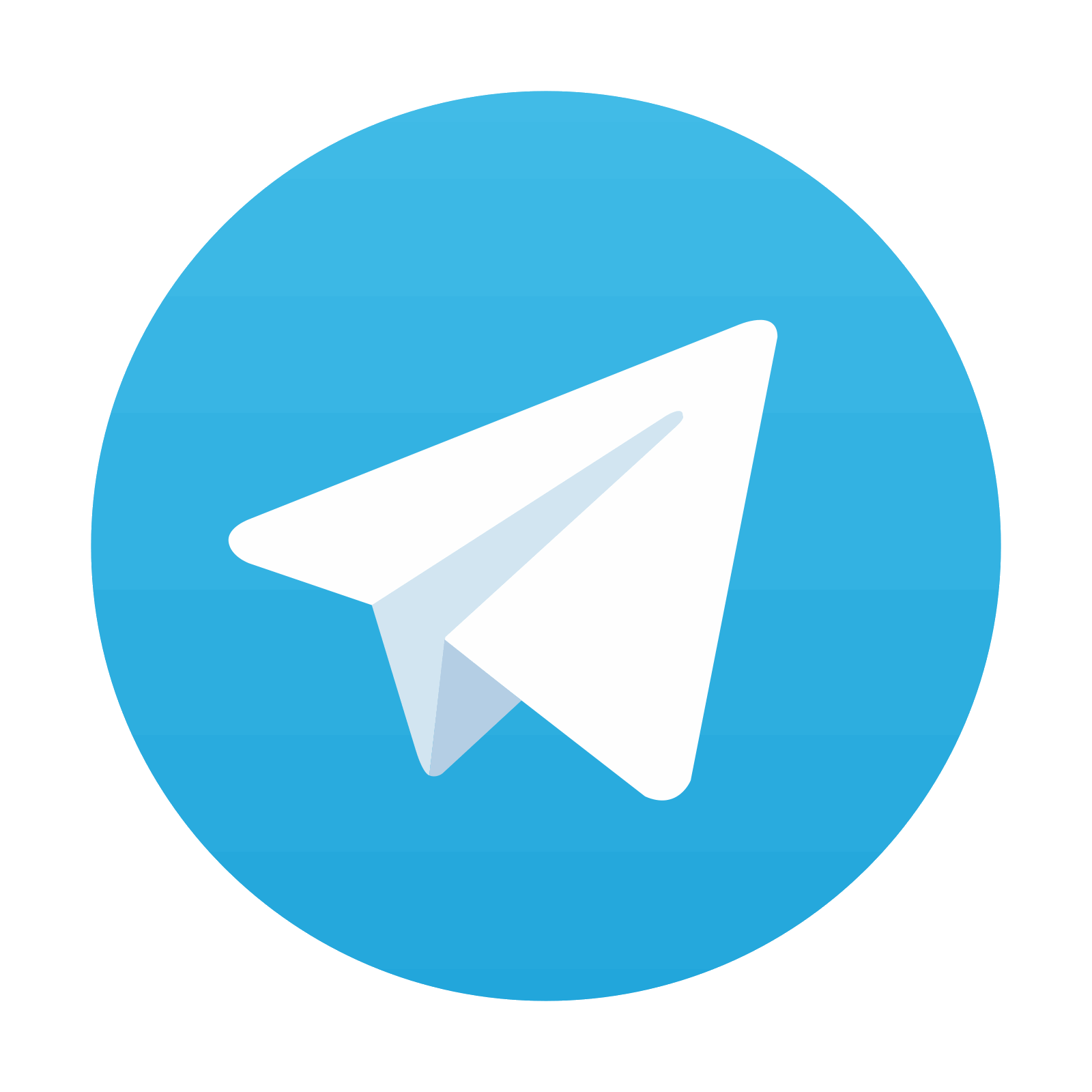
Stay updated, free articles. Join our Telegram channel
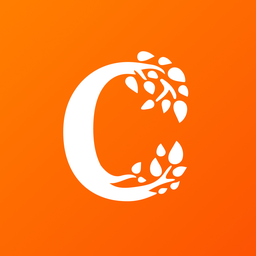
Full access? Get Clinical Tree
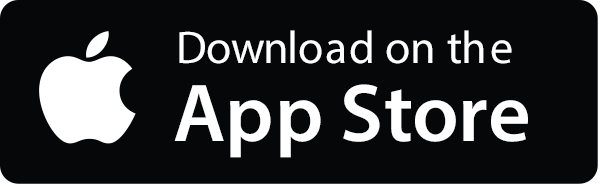
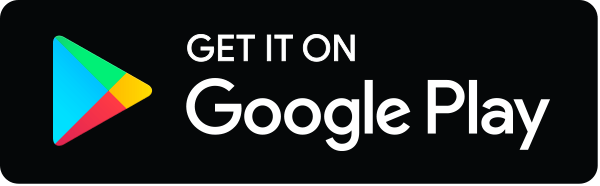
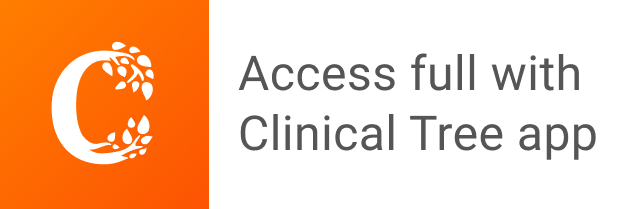