html xmlns=”http://www.w3.org/1999/xhtml”>
John Wiley & Sons, Ltd.
ELS subject area: Evolution and Diversity of Life
How to cite: Wilkins, Jon F (July 2008) Epigenetic Variation in Humans. In: Encyclopedia of Life Sciences (ELS). John Wiley & Sons, Ltd: Chichester.
DOI: 10.1002/9780470015902.a0020811
Advanced article
Epigenetic Variation in Humans
Epigenetic modifications alter the expression behaviour of genes without involving changes to the DNA (deoxyribonucleic acid) sequence itself. These modifications take a variety of forms, most notably DNA methylation and histone modification. Epigenetic differences allow alleles, cells or even individuals that are genetically identical to exhibit radically different phenotypes. Differentiation among cells underlies tissue differentiation and development. Differentiation between alleles within a cell is used to enhance clonal diversity, as in the immune system, and has been highjacked in the service of evolutionary genetic conflicts, as seen in the phenomenon of genomic imprinting. Epigenetic differences among individuals may account for some of the differences between monozygotic (identical) twins. Some recent studies have even suggested that these epigenetic mechanisms may allow organisms to adapt to environmental changes on very short timescales. Environmental adaptations could be epigenetically encoded and passed on to offspring, providing a potential mechanism for a neo-Lamarckian mode of evolution.
Introduction
Epigenetic mechanisms
There is more to a gene than its deoxyribonucleic acid (DNA) sequence. CH Waddington coined the term ‘epigenetic’ to describe biological differences among tissues resulting from the process of development (Waddington, 1939, 1942). Waddington needed a new term to describe this type of variation, which is not the product of genotypic differences among the cells. Neither are these tissue-level differences well described as phenotypic variation, since the variation occurs within an individual organism. We now understand that heritable modifications to the DNA (such as cytosine methylation) and aspects of chromatin structure (including histone modifications) are the mechanisms that underlie Waddington’s ‘epigenotype’. The DNA and its associated proteins are modified in particular cells during development, and those modifications are propagated across multiple cell divisions. Those modifications are responsible for the variation in patterns of gene expression across cell types. In contemporary usage, the term epigenetic refers to any heritable change in gene expression that is not coded in the DNA sequence itself (Egger et al., 2004).
In humans, there are two principal mechanisms of epigenetic modification. The first is cytosine methylation. This occurs overwhelmingly at CpG dinucleotides. These dinucleotides are found in clusters throughout the genome, known as CpG islands. The CpG dinucleotide is a simple example of a ‘pallindromic sequence’. That is, since C pairs with G, a CpG in one strand of the DNA is paired with another CpG in the complementary strand.
The propagation of methylation states across cell divisions depends on this pallindromic structure and the action of the protein Dnmt1, a maintenance methyltransferase, or hemimethylase. The fully methylated form has methyl groups attached to the cytosine on each strand. When the DNA is replicated, these two methylated strands are separated, and new (unmethylated) DNA is synthesized on each. The two daughter cells are then set to inherit hemimethylated DNA (DNA with methyl groups on one strand, but not the other). Dnmt1 specifically recognizes this hemimethylated DNA and attaches a methyl group to the newly synthesized strand. Thus, once the pattern of methylation is set in a particular cell, Dnmt1 acts to propagate this pattern to all of that cell’s descendants.
The second mechanism of epigenetic modification involves various modifications to histones, which are proteins that are physically associated with the DNA. Humans express four distinct histone proteins H2A, H2B, H3 and H4. These histones are subject to a large number of modifications, many of which correlate with the transcriptional state of nearby genes. For instance, H3–K9 methylation (methylation of the lysine at position nine of the H3 histone) is characteristics of transcriptionally inactive heterochromatin. H3–H4 acetylation and H3–K4 methylation are characteristic of transcriptionally active euchromatin.
The stable propagation of epigenetic states across multiple cell divisions relies, in part, on a set of positive feedback mechanisms that link cytosine methylation and histone modification (Wilkins, 2005). The activity of Dnmt1 at a particular hemimethylated site is enhanced both by the presence of nearby DNA methylation and by particular histone modifications. De novo methyltransferases (which act on unmethylated DNA) are also recruited to particular loci by the proximity of these modifications. Likewise, the enzymes responsible for establishing these histone modifications are recruited by the presence of methylated cytosine.
This system of positive feedbacks between DNA methylation and histone modification leads to a kind of ‘epigenetic canalization’. The result is a bistable system in which two states – locally fully methylated and locally fully unmethylated – are each stable with respect to small perturbations. Once methylation is established across a local cluster of CpG dinucleotides, these mechanisms will maintain that methylated state across many cell divisions. For instance, if a single methylation site were to fail to be copied following DNA replication, the existence of neighbouring methylated sites and the presence of modified histones would cause the site to become remethylated at a later time.
The purpose of epigenetic modifications
The genomic DNA sequence encodes the instructions for building the many components that make up a cell. The DNA sequence also includes regulatory motifs, which partially determine when, where and at what level a particular gene product will be produced. It may be best, however, to think of the DNA as encoding the potential for a very broad range of possible expression patterns. Epigenetic modifications provide a kind of filter, which can stably select a particular pattern of gene expression from this possible set. This permits the same genome to stably follow different expression strategies depending on environmental conditions, or to simultaneously exhibit different behaviours in different (genetically identical) cell types in a multicellular organism.
This behavioural differentiation among cell lineages is just one of the roles played by epigenetic modifications. These modifications can also produce differences in the expression behaviour of the two alleles within a single cell. Typically, this involves silencing one of the two copies. This allele-specific silencing is involved in X-chromosome inactivation and parent-of-origin effects, as well as generating variation in the olfactory and immune systems.
DNA methylation and its associated histone modifications also increase the stability of various repeat sequences, including minor satellites, subtelomeric satellites and microsatellites, as well as a variety of repeat-containing transposable elements. Demethylation of these elements results in increased recombination and structural instability of the chromosome (Gonzalo et al., 2006; Guo et al., 2004; Hansen et al., 1999; Kim et al., 2004; Okano et al., 1999; Wang and Shen, 2004; Xu et al., 1999).
The third context in which epigenetic modifications play a crucial role is maintaining these transposable elements in an inactive state. When methylation is lost from transposable elements, those elements become active, and insert additional copies of themselves at random locations throughout the genome, with strongly deleterious effects. In fact, it has been suggested that the ability to protect the genome from these parasitic elements was the primary selective force behind the evolution of our sophisticated epigenetic machinery (Bestor, 2003).
Developmental Epigenetic Variation
Development is the most prominent, and best understood, context in which we find epigenetic variation. By the term ‘developmental variation’, I mean to refer to the variation among cells that is generated during development. This variation is not ‘heritable’ in the sense that it is recreated each generation, and does not necessarily imply heterogeneity among individuals. However, it is heritable within a given cell lineage. This type of epigenetic variation is not the primary focus of this article, but will be discussed briefly here.
Allele silencing
One of the surprising features of the diploid genome is that many genes are expressed from only one of the two alleles. Epigenetic differences between the alleles can generate differences in expression behaviour, even in the absence of any differences at the DNA sequence level.
The most familiar example is likely to be the random inactivation of one of the two X-chromosomes in females. This is commonly interpreted as a mechanism of dosage compensation: males have a single X-chromosome, whereas females have two. Inactivation of one of the two X-chromosomes in females results in similar gene expression levels for X-linked loci in the two sexes.
In some cell types, epigenetic modifications are used to establish clonally stable expression patterns. For instance, several of the interleukins, including IL2, IL3, IL4, IL5 and IL13, are randomly expressed monoallelically in particular subpopulations of T lymphocytes (Bix and Locksley, 1998; Reinhardt et al., 2006). This random inactivation generates a combinatorial assortment of cell lines, each with a distinct, but stable, expression profile. A similar process occurs in the olfactory system, where individual neurons express only one of the order of 1000 distinct odourant receptor genes.
Heritable Variation
The types of epigenetic variation discussed in section Developmental Epigenetic Variation are heritable in two senses. First, these epigenetic states are propagated through multiple cell divisions, and are therefore inherited by the progeny of a particular cell. Second, the epigenetic machinery and the developmental programme that it executes are encoded by the genome. However, neither of these senses implies the concept of ‘heritability’ as it is typically conceived in population genetics. In this section, we will focus on those patterns of epigenetic variation that are passed on from parent to offspring.
Genomic imprinting
In humans and other mammals, approximately 1 per cent of genes are subject to genomic imprinting (Wilkins and Haig, 2003). Imprinting represents a type of allelic silencing, but, in contrast to the forms of allelic silencing discussed in section Developmental Epigenetic Variation, imprinting is the product of epigenetic modifications that are established in the germlines of the parents and inherited by the offspring.
The discovery of the first imprinted genes was precipitated by the discovery that the maternally and paternally derived genomes are not equivalent in mammals. Nuclear transplant experiments showed that gynogenetic embryos (in which both copies of each gene are derived from a female) do not develop into viable offspring. Androgenetic embryos (with two paternally derived genomes) are similarly inviable. In mammals, successful development requires both maternally and paternally derived alleles.
The epigenetic differences associated with imprinted genes are reproduced every generation, and in that way are similar to other forms of developmental epigenetic variation. However, those epigenetic marks are established in the parents and inherited by the offspring.
Most imprinted genes are expressed monoallelically in one or more tissues. This makes these genes particularly susceptible to dysregulation either through mutations in the DNA sequence, or through the loss or reprogramming of epigenetic information. Loss of function or of transcription of the single active allele can result from a single mutation or epimutation. In certain cases, it is possible that such an epimutation may be passed from one generation to another.
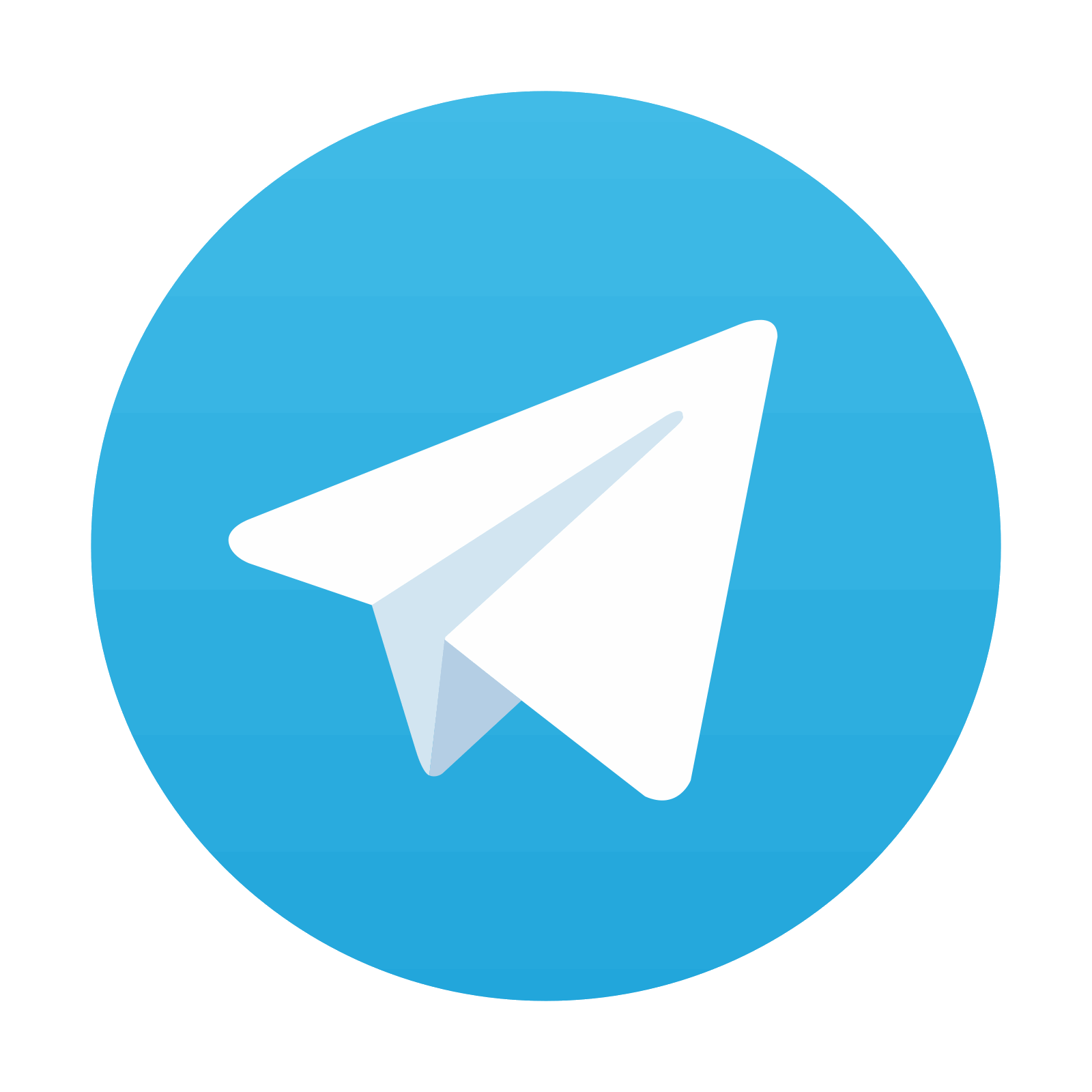
Stay updated, free articles. Join our Telegram channel
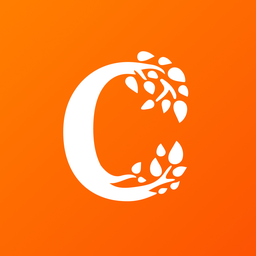
Full access? Get Clinical Tree
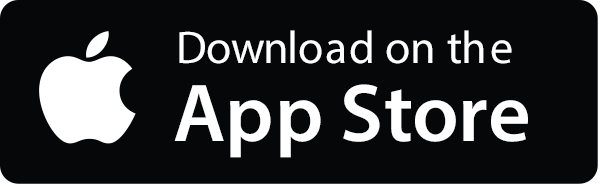
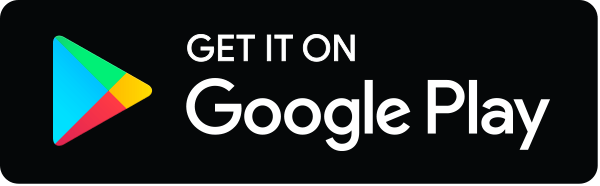