Highlights
- •
The microbiome plays critical roles in immune response to cancer.
- •
Broader efforts are needed to better define the gut and urinary microbiomes.
- •
Microbial metabolites can modulate cancer immunology locally and systemically.
- •
Modulation of the microbiome may improve therapeutics for bladder cancer.
Abstract
Bladder cancer (BCa) remains a significant source of morbidity and mortality. BCa is one of the most expensive tumors to treat, in part because of a lack of nonsurgical options. The recent advent of immunotherapy, alone or in combination with other compounds, has improved therapeutic options. Resistance to immunotherapy remains common, and many patients do not have durable response. Recent advances indicate immunotherapy efficacy may be tied in part to the endogenous bacteria present in our body, more commonly referred to as the microbiome. Laboratory and clinical data now support the idea that a healthy microbiome is critical to effective response to immunotherapy. At the same time, pathogenic interactions between the microbiome and immune cells can also serve to drive formation of tumors, increasing the complexity of these interactions. Given the rising importance of immunotherapy in BCa, understanding how we might be able to alter the microbiome to improve therapeutic efficacy offers a novel route to improved patient care. The goal of this review is to examine our current understanding of microbial interactions with the immune system and cancer with an emphasis on BCa. We will further attempt to define both current gaps in knowledge and future directions that may yield beneficial results to the field.
1
Introduction
Bladder cancer (BCa) is a common solid tumor [ , ]. Treatment of BCa is dependent on stage and grade of the tumor, and in the case of both noninvasive and invasive disease, BCa is routinely treated via immunotherapy [ , ]. High-grade, nonmuscle invasive bladder cancer (NMIBC) is treated with a combination of transurethral resection of bladder tumor and Bacillus-Calmette-Guerin (BCG) immunotherapy, an attenuated strain of mycobacteria that sparks a robust immune response in the bladder to initiate its effects [ , ]. Anti-PD-1/PD-L1 therapy is also approved for use in metastatic BCa. Recent work suggests that combination therapy composed of an antibody-drug conjugate and anti-PD-1 therapy (enfortumab vedotin + pembrolizumab) has promise in treating advanced disease [ , ]. The role of inflammation is likely a double-edged sword though, as persistent inflammation in otherwise benign tissue can drive further tumor progression rather than prevent it [ ]. Understanding the complexities in the interactions between immunity/inflammation and both BCa progression and treatment remains a critical unmet clinical need.
The total human microbiome is composed of a diverse set of microbiota who survive in an array of ecological niches, including the gut, skin, urine, epithelial mucosa and more [ , ]. Microbes interact with cancer through multiple mechanisms and have highly varied effects. A small number of microbes function as causative agents for cancer; however, the majority of microbes could be considered commensal, i.e. they exist harmoniously with the host [ , ]. Commensal microbes are beneficial for cancer surveillance through maintenance of immune training and balance, and mounting evidence suggests the presence of a healthy and diverse microbiome is a major component of immunotherapy efficacy [ , , ]. Microbes and their metabolites are essential components of normal mammalian health and development, so understanding how they impact cancer may further improve our ability to diagnose and treat disease.
As the bulk of all microbial cells is contained in the gut, one of the most commonly discussed microbial niches is the gut microbiome [ ]. In spite of the recent focus on the gut microbiome, the role of fecal or gut bacteria specifically in BCa pathogenesis and treatment remains relatively understudied [ , , ]. In contrast, the urinary microbiome is an area of intense interest in BCa [ , ]. Urine was considered sterile for a significant period of time, but the use of modern sequencing techniques has conclusively demonstrated the persistent presence of bacteria in urine [ , ]. Numerous studies have attempted to define a role for urinary bacteria in BCa progression and treatment, but it has been difficult to reach a consensus due to discordance in the data [ ]. Urine sample acquisition, storage, timing and other variables likely complicate these studies; moreover, the site of acquisition in the urinary tract may complicate repetition of studies [ ]. A better understanding of how the urinary and fecal microbiomes can alter progression and therapy in BCa is needed.
The purpose of this review is to understand how interactions between microbes and the immune system can ultimately impact BCa development and response to treatment. We will define mechanisms of how microbes interact with immunity and explore the gut and urinary microbial constituencies in BCa as they are currently understood. Finally, novel potential mediators of these interactions will be identified in addition to noting exciting areas where we hypothesize further progress can be made that would benefit the field.
2
Microbial interactions with the immune environment and cancer
2.1
Microbial-immune interactions
The human microbiome contains diverse microorganisms including bacteria, viruses, fungi, and more, with an overwhelming majority of microbiota thought to be found in the gut [ ]. Accordingly, the gut-immune axis will be highlighted as a model system with potential means for altering disease course and enhancing therapeutic efficacy.
The primary function of the immune system is to remove pathogenic material and prevent infection. This requires a careful balance between immune function to prevent disease and immune tolerance to maintain host health without the development of autoimmunity. Maturation and education of the immune system occurs relatively early in life and is dependent on interactions between gut bacteria and the immune system [ ]. The gut microbiome is known to undergo significant changes over time related to diet, environmental exposures, aging and other factors, but gut bacteria continue to shape immune function throughout life [ , , ].
Bacteria in the gut functionally alter immunity through both local and distant interactions. Bacteria express p athogen or m icrobial associated molecular patterns (PAMPs/MAMPs), which represent a diverse array of proteins, nucleic acids and bacterial structural elements, such as lipopolysaccharide (LPS) [ , ]. PAMPs and MAMPs are recognized by intracellular and extracellular pattern recognition receptors (PRRs) on antigen presenting cells (APCs). Dendritic cells and macrophages are examples of phagocytic APCs that respond to these signals through activation of pro-inflammatory immune signaling cascades [ , ]. ( Table 1 ) Signaling cascades initiated by PRRs results in initiation of an immune response through the upregulation of pro-inflammatory cytokines such as interferon gamma (IFN), tumor necrosis factor alpha (TNF-α), and pro-inflammatory interleukins via transcription factors including nuclear factor kappa B and activator protein-1 [ , ]. These interactions are further regulated by cellular signaling between innate immune populations and multiple sets of adaptive immune cells including regulatory T cells and CD8 + T cells to further hone this response [ , ]. While this is normally a homeostatic interaction that is critical to immunity and immune tolerance, alterations in microbial content can sway this balance to either autoimmunity or reduced immune function resulting in increased risk of infection. In the case of cancer, gut-immune interactions are critical to recruit and activate anti-tumorigenic T cells [ , , ]. The anti-tumorigenic effects of microbes, such as those in the Bifidobacterium genera , Akkermansia muciniphila and Enterococcus hirae , have been directly linked to function at least in part through increased CD8 + T cell activity [ , , ].
Receptor | Associated PAMP/Ligand | Cellular Location |
---|---|---|
TLR2 | peptidoglycans | Cell Surface |
TLR3 | viral dsRNA | Cell Surface |
TLR4 | LPS | Cell Surface |
TLR5 | bacterial flagellin | Cell Surface |
TLR7 | ssRNA | Cell Surface |
TLR9 | unmethylated CpG DNA | Cell Surface |
NOD1 | peptidoglycans | Intracellular |
NOD2 | muramyl dipeptide | Intracellular |
CD206 | Mannose repeats, N-acetylglucosamine, sugars | Cell Surface |
Bacteria also secrete many different compounds that can potentially alter immune function systemically. PAMPs and MAMPs can become systemically available, particularly in cases of pathophysiological changes in the gut that increase gut permeability [ , ]. As mentioned, PAMPs and MAMPs activate PRRs on diverse cell types and can alter immune signaling and function [ ]. Moreover, bacteria also secrete extracellular vesicles (EVs) or outer membrane vesicles (OMVs) that contain these same immunogenic components which can function as signaling agents [ ]. Aside from PAMPS or MAMPs, bacterial metabolites such as butyrate and other short chain fatty acids (SCFAs) can reduce immune function through suppression of T cell activity [ , ]. The role of SCFAs is complicated, though, and likely involves induction of contextual inflammatory signals through interactions with colonic and systemic regulatory T cells in addition to CD8 + T cells [ ]. Bacteria also produce an array of bioactive compounds that can potentially alter cancer formation which are discussed in more depth in Section 3 .
Perhaps the best evidence for interactions between the gut microbiome and immunity come from studies using germ-free (GF) animals or animals/patients treated with antibiotics that deplete gut bacteria [ , , ]. Loss of gut-immune interactions in GF animals reduces formation of de novo cancer in models of liver, colon, and lung cancer via reduced pro-tumorigenic inflammatory activation [ ]. Other models have demonstrated increased tumor formation in GF mice or a lack of change between conventional and GF mice, which potentially illustrates a more predominant role for early inflammation in promotion of tumor formation in a specific subset of tumors [ , ]. Studies in a genetically engineered K-ras driven model of lung cancer demonstrate that a loss of commensal microbiota in the lung reduces production of gamma delta T cells that stimulate inflammation and exacerbate tumorigenesis [ ]. Gut commensal microbiota are thought to enhance liver cancer induced by the carcinogen diethylnitrosamine through LPS:TLR4 dependent signaling that promotes inflammation and drives tumor formation through increased production of epiregulin and hepatic growth factors [ ]. Definitive evidence for how either gut or urinary/bladder mucosal microbiota contribute to mouse models of BCa is currently limited though. Although there are established murine models of BCa, such as genetically driven models or the N -butyl- N -(4-hydroxybutyl) nitrosamine BBN driven model, studies of the microbiome using these models are lacking, although it would be beneficial to understand how loss of microbiota affects tumor formation in these models [ , ].
While loss of gut bacteria can reduce cancer formation, recent high profile studies have demonstrated a very different role for commensal bacteria when treating already developed cancers [ , , , , , ]. GF animals commonly do not respond to chemotherapy or immunotherapy as well as conventionally grown animals as they generate a less potent immune response [ , , ]. Similarly, administration of antibiotics to deplete gut bacteria reduces the efficacy of immune checkpoint inhibitors in multiple cancers [ , ]. This is supported by clinical studies indicating patients that receive antibiotics prior to or during immunotherapy have poorer outcomes in multiple tumor types, including tumors outside the gut, such as BCa [ , , , , ]. Furthermore, recent data in multiple mouse models indicates specific microbial components can support tumor immunity [ , , ]. Bacteria such as Akkermansia muciniphila, Enterococcus hirae, and Bifidobacterium , are associated with increased effectiveness of immunotherapy as demonstrated by clinical trials that suggest the presence of the “right” amounts of these bacteria is associated with therapeutic response in patients [ , , , ]. In support of these data, high-fiber diets that enrich the microbiome with beneficial species may be capable of enhancing immunotherapy in murine models by converting the underlying tumor microenvironment towards a more anti-tumorigenic phenotype [ ]. High-fiber diets promote accumulation of bacteria that are associated with increased production of cyclic dinucleotides that activate the stimulator of interferon (STING) pathway and may ultimately enhance immunotherapy efficacy [ ]. Human trials support the idea that high-fiber diets can provide greater immunotherapy efficacy, but recent work has further noted that this effect is more complex than fiber simply being beneficial, and moreover, concurrent use of pro-biotics may actually dampen therapeutic efficacy even when patients have sufficient fiber intake [ , ]. Dietary interventions may be a viable methodology for increasing the likelihood of immunotherapy efficacy, but selective uptake of specific strains of bacteria has been difficult to sustain in murine models without prior antibiotic treatment, thus methodology to achieve this should be carefully validated.
Another option for improving the gut microbiome is through fecal microbiome transplantation (FMT). FMT offers the potential for transplanting an entire microbiome that has already been associated with clinical response [ ]. Early stage clinical trials have proven FMT is safe in cancer patients and have demonstrated early signs of anti-tumorigenic clinical activity in a small subset of patients [ , ]. Given the massive number of organisms present in the body, it could be speculated that gut-immune interactions are highly dependent upon the overall composition of the microbiome, rather than any single individual group, but some species still may be useful for shifting the balance towards a specific goal [ , , ]. It should also be noted that microbial interactions with cancer treatment efficacy are not limited exclusively to immunotherapy. Efficacy of treatment with cyclophosphamide or oxaliplatin, a platinum salt related to cisplatin, was linked to the underlying microbiome as well [ , ].
Although microbes are largely beneficial, pathogenic microbes can also invoke tumor formation. The presence of persistent, uncontrolled inflammation in otherwise benign tissue is associated with tumor formation [ ]. Infection with a number of microbes, such as Hepatitis B/C, Helicobacter pylori, and schistosomes cause persistent inflammation, which can induce or promote tumor formation directly [ ]. Thus, unresolved inflammation is typically undesirable, particularly in otherwise benign tissue. In contrast, current immunotherapy treatments generally result in cycles of effective inflammation that self-resolve. For instance, immunotherapy with BCG does not result in BCG being permanently incorporated into the underlying microbiome, and instead yields repeated cycles of anti-tumorigenic inflammation followed by immune resolution [ ].
In summary, these studies illustrate how interactions between the microbiome and the immune system are highly dependent on the context of the cancer and the underlying microenvironment. Inflammation can be either beneficial or detrimental to overall outcomes and should be considered in the context of the current state of the cancer and cancer therapy. Current data strongly support the hypothesis that the microbiome is critical to cancer progression and cancer therapy. Further understanding how the microbiome interacts with traditional chemotherapy may yield actionable targets for improving therapy or defining suitable patients. Defining these roles in BCa specifically is an unmet need in the field.
3
Microbial metabolites and other potential systemic mediators of microbial action on cancer development and cancer immunity
3.1
Microbial metabolites
Commensal microbes express a vast array of metabolic enzymes that assist in digestion and metabolism in the body [ ]. Some of these are not otherwise produced by mammalian cells [ ]. Microbial metabolites have critical roles in tumor-associated processes including cellular proliferation, inflammation, chemotherapy efficacy and more [ ]. We will briefly discuss potential roles for bacterial metabolites and bacterially derived mediators in the development of BCa. Notably, the majority of these mediators are produced predominantly in the gut; however, when possible, we will note the potential for similar actions to occur in the bladder although experimental evidence demonstrating the contribution of bladder or urinary microbiota to these processes are limited.
3.2
Short chain fatty acids
SCFAs are bacterially derived nutritional metabolites that function as a major food source for colonocytes in the gut, serve as ligands for free fatty acid receptors, and support epithelial barrier integrity [ ]. SCFAs such as butyrate can also modulate inflammation, complicating their role in cancer. In vitro , butyrate alters inflammation as exposure to butyrate blocks IL-10 and monocyte chemoattractant protein-1 (MCP-1) production and enhances prostaglandin E2 production in LPS-exposed monocytes [ ]. In vivo, systemic levels of butyrate are associated with reduced efficacy of CTLA-4 mediated therapy in melanoma, however, this finding required continued exposure to water with very high concentrations of butyrate (100mM), which only modestly increased serum butyrate levels [ ]. Nevertheless, loss of CTLA-4 response was attributed to the higher concentrations of systemic butyrate preventing upregulation of co-stimulatory molecules CD80 and CD86 [ ]. Serum SCFA levels, including butyrate and propionate, are also associated with increased regulatory T cell accumulation, consistent with prior papers correlating increased colonic and peripheral regulatory T cell formation with SCFA administration [ , ]. SCFAs also have direct anti-tumorigenic activity in tumor cell lines, including BCa [ ]. One of the primary proposed mechanisms for butyrate’s anti-tumorigenic activity is through inhibition of histone deacetylase activity [ ]. Inhibition of histone deacetylase activity alters gene expression to reduce cellular proliferation in vitro at higher concentrations of butyrate [ ]. Notably, lower concentrations of butyrate can stimulate cell proliferation and increase histone acetylase activity; although this is dependent on cellular metabolic factors including activation of the Warburg Effect [ ]. The anti-tumorigenic activity of butyrate and other SCFAs is variable between cells, but typically requires millimolar levels of butyrate in vitro to halt proliferation in BCa cell lines [ , ]. It is unlikely systemic levels—which are typically micromolar— reach the concentrations necessary to directly limit tumor formation in the bladder unless there is significant accumulation of SCFAs intracellularly or local production of SCFAs [ ]. If there is substantial production of butyrate by bladder associated microbiota this could yield pathophysiologically relevant concentrations of butyrate, although this hypothesis is untested in the literature to the best of the author’s knowledge.
SCFAs are minimally excreted into urine (<1%) but in spite of minimal excretion, prior research reports acetate levels of up to 130µM in healthy subjects, although this is highly variable [ , ]. Because of the minimal excretion into urine and the reasonably high concentrations of acetate in some patients, it is tempting to hypothesize that SCFA levels in urine may be produced directly by bacteria in the urine or bladder. SCFA producers such as Bacteroides and Akkermansia have been reported in bladder tissue [ ]. High levels of these bacteria in the bladder may ultimately impact SCFA levels locally in the bladder, although direct evidence for this hypothesis is still lacking in the literature. The presence of high quantities of butyrate-producing microbes in the gut or bladder may have differential effects in various tumor types, particularly in the context of CTLA-4 immunotherapy though. A prior study noted that BCa is associated with a modest increase in serum butyrate levels in a small population, however, understanding whether or not high systemic levels of butyrate portends poor results with chemotherapy or immunotherapy in BCa remains an open question [ ].
3.3
Polyamines, amino acids, and other microbial metabolites
Polyamines including putrescrine, spermidine, and spermine are present in all mammalian cells and are required for life. Polyamines are made by both mammalian cells and bacteria. While endogenous production of polyamines is thought to be the dominant source of most cellular polyamines, bacterial production of polyamines may affect fecal or systemic levels [ ]. Increased levels of polyamines and polyamine metabolism is tightly associated with cancer, in addition to cancer-related processes like autophagy and aging [ , ]. Inhibition of polyamine metabolism by the ornithine decarboxylase inhibitor eflornithine reduces tumor outcomes in mouse models of BCa, but this is likely primarily through inhibition of mammalian polyamine metabolism [ ]. Polyamines are present in both bladder tissue and urine, but the contribution of the microbiome to these levels is not well understood [ , ]. Bacteria are broadly capable of synthesizing polyamines and may contribute to overall polyamine levels, particularly in the gut where spermidine and putrescine levels are linked to the presence of gut microbes [ ]. A better understanding of their contribution to polyamine synthesis in the urinary tract is needed and could be pursued through the use of GF animals. Similarly, high-protein diets are associated with increased levels of bacterial products, such as p- cresol and N-nitroso compounds, and may be linked to BCa and other pathologies [ , ]. Specifically, N- nitroso compounds, such as the model carcinogen BBN, are known to induce BCa [ ]. Finally, the gut is known to heavily influence metabolism of dietary amino acids such as tryptophan, resulting in the production of intermediates of the kyneurine pathway, serotonin, and indoles that serve as agonists of the aryl hydrocarbon receptor [ ]. All of these molecules can affect signaling pathways both in cancer cells and the underlying tumor microenvironment [ ]. The potential that specific microbiomes could increase systemic levels of bacterial metabolites that ultimately accumulate in the bladder and alter BCa remains largely untested, in part because of the low number of studies identifying the gut microbiome of BCa patients. Similarly, because of the relatively basic understanding of the urinary microbiota versus tissue microbiota, there is minimal understanding of the ultimate impact of these bacterial groups on tissue levels of relevant compounds.
3.4
Microbial extracellular vesicles and outer membrane vesicles
In addition to an array of metabolites, bacteria also produce and secrete EVs. EVs are lipid bound small bodies secreted by both mammalian and nonmammalian cells that contain various cargo from the originating cells [ ]. Recent evidence suggests bacteria produce large quantities of EVs, including OMVs derived from Gram-negative bacteria [ ]. These vesicles contain bacterially derived nucleic acids, proteins, and structural components of the bacterial cell wall, all of which can function as potent immune modulators [ ]. Bacterial EVs and OMVs reach systemic circulation where they may broadly alter immune signaling depending on the context of their cargo, such as the presence of virulence factors or PAMPs/MAMPs [ , ].
EVs or OMVs from different bacteria have been found to have potential therapeutic use. In 1 study, OMVs were isolated from an E. coli strain with a genetically inactivated lipid A acyltransferase gene that produces a form of LPS in OMVs that cannot directly interact with TLR4. These OMVs were given systemically to mice bearing colon tumor xenografts and were found to have direct anti-tumorigenic effects through increased IFN production, as IFN-deficient mice did not respond to OMV mediated therapy [ ]. Perhaps more remarkably, EVs from either Gram-positive Lactobacillus acidophilus or Staphylococcus aureus also had a therapeutic effect against colon cancer xenografts, indicating bacterial EVs or OMVs may have a broad capacity to induce immunity [ ]. Akkermansia muciniphila is a Gram-negative bacteria associated with effective immunotherapy in patients when present at the appropriate relative abundance [ , ]. Recent evidence indicates extracellular vesicles from A. muciniphila can alter immunity through increased recruitment of M1 macrophages and increased CD8 + T cell activity in prostate cancer xenografts [ ]. Other studies have implicated the complexities of the extracellular cargo as important given that A. muciniphila EVs contain an acetyltransferase that could functionally alter histone acetylation and lead to reductions in colon tumor formation [ ].
Although it remains untested, it may be possible to use bacterial EVs or OMVs to improve BCa therapy. Of note, EVs derived from BCG were found to have similar attributes to BCG itself in vitro [ ]. As BCG shortages can affect access to therapy, EVs may ultimately provide a stopgap or alternate means of treating patients if they truly recapitulate the entire response, but this still needs to be initially tested in murine models. Perhaps most notably with regards to BCa, Firmicutes -associated DNA was associated with response to pembrolizumab in patients with BCa, as patients with high levels of Firmicutes DNA in EVs had poorer response to pembrolizumab and fewer infiltrating T cells [ ]. As noted above, the gut contains the bulk of all bacteria in the body. The contribution of systemic EVs or OMVs as compared to locally produced bacterial EVs or OMVs is not well understood, particularly in the context of BCa. Current technology to differentiate microbial versus nonmicrobial EVs is still somewhat limited, but is continuing to evolve [ ]. The development of highly robust methodology to rapidly and selectively evaluate origin and cargo of EVs in vivo would be highly beneficial to broadening our understanding of the role of endogenous bacterially derived EVs.
In summary, a number of microbial metabolites and microbial derived signaling mediators can ultimately affect cancer processes through a diverse array of mechanisms. These factors largely remain underexplored in the context of BCa. Future studies attempting to evaluate these factors in the context of the microbiome could provide mechanistic data to support findings.
4
Microbiomes and BCa
Numerous studies have demonstrated that changing the underlying gut microbiome can sufficiently alter immune function enough to affect immunotherapy or cancer outcomes in varied tumors including lung, liver, melanoma, and more [ , , , , , ]. There have been relatively few investigations of the role of the gut microbiome and its interactions with immunotherapy with direct regards to BCa. Furthermore, it is now well understood that urine is not sterile, and in fact persistently harbors commensal bacteria [ , ]. Given the evidence that microbial actions on tumor can also happen through local, commensal bacteria, urinary microbiota may be critical to understanding BCa development and treatment [ ]. Novel studies defining any potential roles for the microbiome in BCa are imperative for advancing the field.
4.1
The urinary microbiome and BCa
4.1.1
Constituency
The primary physiological role of the bladder is storing and expelling urine. Recent advances have definitively demonstrated a native, commensal population exists in urine, but attempts at identifying the core urinary microbiome is an ongoing and multifaceted challenge. Difficulties stem from the characteristics of the urinary microbiome, such as having a relatively low biomass in comparison to the gut microbiome and its proximity to gut and vaginal sites, increasing the possibility of sample contamination [ ]. Collection techniques vary as well and yield differing results as voided samples may be more representative of urethral microbiomes, while catheterized samples are more reflective of the bladder itself [ ]. Urinary dysbiosis, or detrimental changes to the urinary microbiome, is observed in both genitourinary and systemic disease states. Various systemic pathologies, such as diabetes, hypertension, and chronic kidney disease, have been associated with urinary dysbiosis, as well as antibiotic use that may result in decreased abundance [ ]. Characterizing the effects of dysbiosis have been made more difficult in part by the lack of cohesiveness on which microbiota constitute the core members of the urinary microbiome.
Various studies have proposed a composition for the urinary microbiome, which is further stratified based on sex and age. Firmicutes and Proteobacteria have been identified as predominating phyla, but there are differing results of the predominate genus [ , ]. It was previously suggested that Corynebacterium was most abundant in males, however, newer literature proposed that Streptococcus is the most abundant [ , ]. Furthermore, it has been shown that the Lactobacillus genus is the most abundant in females, and their urinary microbiome has more bacterial diversity with a median genera of 21, compared to 11 in male samples in 1 study [ ]. Age-based differences have been previously explored, with midstream urine samples of individuals greater than 70 years of age having a greater abundance of Jonquetella, Parviomonas, Proteiniphiul, and Saccharofermentans [ ]. A newer study comparing the urinary microbiome in 34 pre and 20 postmenopausal women found that postmenopausal women have greater α-diversity and abundance of Gardnerella, Prevotella, and Escherichia-Shigella [ ]. Although Lactobacillus is the most abundant genus in both female groups, the relative abundance is less in the postmenopausal group, posing as a potential marker of age-related dysbiosis [ ]. Given well known sex differences in BCa incidence, these changes may contribute to underlying pathology, although no direct link has been formed. Identifying markers of dysbiosis is relevant to the field as there is an evolving interest in the role of urinary dysbiosis in genitourinary malignancies.
Various markers of urinary dysbiosis in the context of BCa have been proposed. Using data from 3 previous studies, Bukavina et al. 2023 broadly found urine samples of the BCa cohorts to be associated with polycyclic aromatic hydrocarbon (PAH)-degrading bacteria, notably Sphingomonas [ ]. PAHs are relevant to BCa as there have been 16 identified PAHs in tobacco smoke, which is a risk factor for BCa development [ ]. These findings suggest that degradation products of tobacco may alter the urinary microbiome, favoring bacteria that can facilitate the breakdown of PAHs. Additional studies support these findings, as demonstrated in a 2b Restriction-site Associated DNA tag sequencing for microbiome (2bRAD-M) of BCa tissue where another PAH-degrading species, Ralstonia , had the highest abundance in both MIBC and NMIBC [ ]. Interestingly, NMIBC samples had increased diversity and relative abundance of Acinetobacter, Brevibacillus, Staphylococcus species, unlike MIBC where Ralstonia mannitolilytica and picketti dominated [ ]. Since the microbiomes of the NMIBC cohort had increased diversity, it is possible that maintenance of microbial diversity is a protective factor against disease progression. Overall, there are mixed results on which species are most abundant in BCa, as there is evidence for increased Actinobaculum, Porphyromonas, Propionimicrobium, Veillonella, and Methylobacterium species [ , ]. Contradictory data is also present when comparing the diversity of BCa microbiomes to control patients, with varying conclusions on α-diversity, although this is likely mediated in part by collection methods [ , , ]. Based on previously mentioned challenges and the multitude of techniques to assess the urinary microbiome, the mixed results are unsurprising and warrant further confirmatory studies using clearly defined metrics and multiple collection sites. Factors such as the site from the samples was taken, sex, diet, and age may need to be more tightly controlled to generate consistencies or define baseline differences.
Analysis of microbial richness derived directly from bladder specimens indicates potential differences between urine and the bladder tissue microbiome, but this also may be dependent on sample site. One study indicated that the most common phyla was Proteobacteria, followed by Bacteroidetes and Firmicutes in both cancerous and noncancerous tissue, similar to what has been observed in urine [ ]. This study was able to further stratify cancerous versus noncancerous tissue through analysis of the bladder tissue microbiome with a high degree of certainty using random forest predictive models (Receiver Operator Characteristic = 0.93) [ ]. Microbial constituency within bladder tissue itself differs from the urinary microbiome, contingent on where urine is acquired from though [ ]. Analysis of ß-diversity from 21 men and 8 women indicates urine or non-neoplastic tissue and neoplastic bladder tissue clustered differently when compared from the same set of patients [ ]. This urine was acquired from voided samples that could potentially lead to enrichment of lower urinary tract species, but the findings still suggest a contextual difference between urine and bladder tissue [ ]. In contrast, a separate study found that catheterized urine acquired directly from the bladder during tumor resection showed high levels of similarity between tissue and urine samples, although this study also found that male and female samples bladder tissue samples were significantly different than one another when assessing observed richness and Shannon diversity at the genus level [ ]. Interestingly, a few genera were overrepresented at the tissue level in this study including Akkermansia, Bacteroides, Clostridium, Enterobacter and Klebsiella [ ]. Bacteroides and Akkermansia both use and degrade mucin as an energy source, and thus may reduce the mucinous barrier between urothelial cells and urine [ ]. Loss of the mucinous barrier could increase exposure to xenobiotics or enhance the inflammatory effects of exposure to urinary bacteria, both of which could potentially enhance carcinogenesis. As noted above, though, Akkermansia is often associated with therapeutic response, which may complicate results [ , , ]. Studies testing the underlying role of tissue versus urinary microbiota may be warranted based on these findings. Moreover, clear delineation of voided versus catheterized urine is likely needed to discuss findings in the context of BCa [ ].
4.1.2
Impact of the urinary microbiome on BCa
The impact of the urinary microbiome on BCa development and therapy is an area of particular interest to the field. One particularly notable pathologic change is schistosome infection, or schistosomiasis, where chronic inflammation secondary to production of N-nitrosamine acts a major risk factor for squamous cell carcinoma (SCC) of the bladder [ ]. To date, there is a lack of literature specifically evaluating the urinary microbiome of individuals with known schistosomiasis and subsequent BCa; however, 1 study observed that Sphingobacterium is in higher abundance in the schistosomiasis infection group compared to controls [ ]. Thus, Sphingobacterium may act as a marker for schistosomiasis infection, or potentially have a role in disease as ceramides produced by Sphingobacterium can activate macrophages and provoke subsequent inflammation [ ]. Schistosomiasis is thought to lead to chronic innate immune stimulation, which is implicated as potentially tumorigenic in multiple cancers [ , ]. Similar to schistosomiasis, the presence of multiple urinary tract infections predisposes towards increased risk for SCC of the bladder, likely through persistent spikes in innate immune activity [ , ]. While SCC is a much less common form of BCa than the more typical urothelial cell carcinoma or transitional cell carcinoma, these data support the idea that SCC may be more tightly linked to persistent infections than other forms of BCa. A greater understanding of urinary dysbiosis from varied causes is needed to determine if similar occurrences happen prior to BCa in the absence of overt infection.
Recurrence and progression are common concerns with high-grade NMIBC, even when patients are treated with BCG. Understanding the urinary microbiome in the context of BCG treatment is potentially important as it may offer biomarkers of response or methods to increase therapeutic efficacy. A recent study assessing the urinary microbiome before BCG induction and again directly prior to intravesical treatment concluded an overall decrease in richness and that NMIBC patients without recurrence exhibited less α-diversity and greater amounts of Ureaplasma and Escherichia – Shigella species, while the Aerococcus genus was increased in the recurrence group [ ]. Further demonstrating the difficulty in assessing the urinary microbiome, a 2024 study indicated minimal difference in the urinary microbiome after BCG therapy when intermediate/high risk patients were compared to control patients with benign prostatic hyperplasia [ ]. This study assessed the urinary microbiome directly prior to BCG treatment and at multiple time points prior to BCG instillation during the overall course of treatment. Since these measurements were made from catheterized urine samples acquired immediately prior to BCG instillation, they may yield different results than samples acquired after BCG instillation [ ]. Anti-PD-1 or anti-PD-L1 therapy may also ultimately be affected by the urinary microbiome, but there is currently minimal data in this arena. PD-L1 expression was linked to the presence of the underlying microbiome in a small cohort of patients [ ]. Additional studies are needed to explore these relationships in larger multicenter trials with consistent sampling methodology through a variety of time points.
The efficacy of treatment may also be altered by concurrent antibiotic use, as both the urinary and gut microbiomes can be affected by antibiotics. In a retrospective study in NMIBC patients who had undergone a regimen of transurethral resection of the bladder followed by BCG, the 5-year recurrence free survival and progression-free survival was increased in patients who had not received concurrent antibiotics at the time of BCG compared to those who had received a course of antibiotics for ≥ 7 days [ ]. As noted previously, it is increasingly well established that antibiotics can affect PD-1 therapy in the context of urothelial cancer and others [ , , , ]. Recent studies have further indicated that specific antibiotic regimens may affect PD-1 therapy differently as antibiotics have varying effects on the expression of mucosal vascular cell adhesion molecule-1 (MADCAM-1), which was ultimately found to correlate with immunotherapy efficacy [ ]. The vast differences in drug disposition and spectrum of activity of antibiotics may affect which microbial communities are ultimately implicated in immunotherapy differences. For example, oral administration of vancomycin limits its bioavailability largely to the gut, whereas nitrofurantoin is thought to accumulate in the urinary tract to initiate antibiotic therapy. Laboratory experiments targeting specific compartments of the body with antibiotics that have exclusivity in their distribution may offer a means for discerning these roles.
It is notable that the preponderance of evidence supporting a role for general dysbiosis (i.e. dysbiosis exclusive of an infection with a known oncogenic agent) as a direct driver of cancer formation is most typically found in colorectal cancer (CRC). The gut microbiome of CRC patients looks substantially different from the gut microbiome of non-CRC patients including increased abundance of Bacteroides, Escherichia, Fusobacterium and Porphyomonas , and transplant of feces from CRC patients promotes intestinal carcinogenesis, even in GF mice [ , ]. In contrast, there is minimal evidence in favor of generalized dysbiosis of the urinary microbiome as being directly responsible for the development or progression of BCa in the absence of schistosomiasis infection. This is limited in part by difficulties in defining the true urinary microbiome, and also because while FMT is highly achievable, there are no current reports of transplant of the urinary microbiome to the best of the author’s knowledge [ ]. Solidifying our understanding of the underlying microbiome and developing tools to selectively alter it would be highly beneficial to dissecting the role of the urinary microbiome in tumor formation and treatment.
4.2
The gut microbiome and BCa
4.2.1
Constituency in BCa
The gut microbiome has been extensively studied in the past decade, particularly with regard to its role in host immunity. The normal gut microbiome is composed of a plethora of bacteria, including Clostridia, Bacilli, Flavobacteria, Bacteroidia, and Sphingobacteria [ ]. Associated with gut dysbiosis is concomitant increased permeability of the endothelial gut barrier that has been implicated in both dysbiosis and other disease states [ ]. A potential causal relationship exists where either dysbiosis results in increased gut permeability or increased gut permeability results in dysbiosis, but the causality of this relationship is an area of uncertainty. Regarding BCa, in a genome-wide association study there is a significant association of increased risk of BCa with Bifidobacterium, Actinobacteria , and Ruminococcustorques species [ ]. The association of increased risk with Bifidobacterium is interesting as others have associated Bifidobacterium with therapeutic efficacy [ ]. Other fecal DNA analysis noted a decrease in Clostridium, Prevotella, Lactobacillus, and Bifidobacterium in BCa patients, as well as Roseburia , which notably produces butyrate [ , ]. Decreased fecal SCFA levels and increased LPS were also found in a small population of patients with BCa [ ]. Altogether, these new findings characterize a potential relationship between gut dysbiosis, barrier permeability, and BCa, but no causal evidence exists as of yet. It is important to note that there is a paucity of data in the literature that directly test this hypothesis. Large, multicenter trials with long-term outcomes associating gut microbial changes with BCa are lacking. Examining the relationship between urinary and gut dysbioses in terms of causality of BCa remains imperative, as does understanding if gut dysbiosis ultimately affects urinary dysbiosis or vice versa.
An overview of potential interactions in the bladder between cancer cells, microbes, microbial metabolites and the immune system is depicted in Fig. 1 .
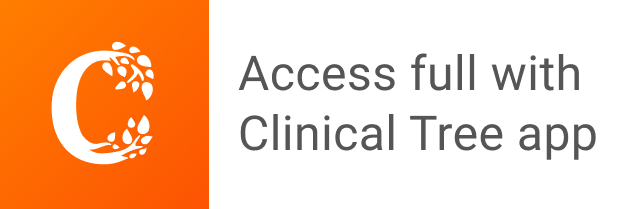