If normal values are substituted in the equations above, a healthy adult male of 70 kg body weight will have an O2 delivery of about 1000 ml/min. His basal O2 consumption at rest, which is given by the equation:
and will be about 250 ml/min. Fortunately evolution has blessed the O2 delivery system with substantial reserve capacity. Provided that the cardiac output and arterial O2 saturation are maintained within the normal range, a fair degree of anaemia can be tolerated.
There is some evidence that O2 delivery may be enhanced by a moderate degree of acute normovolaemic anaemia. In dogs rendered acutely anaemic by the withdrawal of blood, and kept normovolaemic by the simultaneous infusion of dextran, calculated O2 delivery peaked at an Hb level of 100 g/l, and fell below the pre-phlebotomy level at an Hb level of about 85 g/l. The apparent improvement in O2 delivery was attributed to a fall in blood viscosity and a doubling of the cardiac output (Messmer et al. 1972). Subsequent human studies appeared to confirm these findings (Messmer 1987). However, Weiskopf and co-workers (1998), in a careful study of non-medicated volunteers, measured no rise and peak in O2 delivery as the Hb declines and found that O2 delivery begins to fall at a lower Hb value that is age and sex dependent (males = 70 g/l; females = 60 g/l). At lower Hb levels, when O2 content is reduced, demand for O2 is met by increased extraction of O2. The mixed venous O2 content then falls (Wilkerson et al. 1987). In chronic normovolaemic anaemia, the low arterial O2 content is further compensated by a shift of the O2 dissociation curve to the right.
Some organs normally extract more O2 from the blood that perfuses them than do others. Although the whole body arteriovenous O2 content difference is normally 5 ml/dl at rest, corresponding to an extraction ratio of 25%, the heart normally extracts 55% of the O2 from the blood it receives and the brain 35–40%. Substantial increases in coronary and cerebral blood flow will therefore be required to maintain O2 delivery to the heart and brain in the presence of normovolaemic anaemia. In normovolaemic humans, cerebral blood flow rises when the PCV is reduced to 0.28 (Paulson et al. 1973). The reduction in blood viscosity that results from haemodilution of this order appears to be beneficial in patients with focal cerebral ischaemia (Kee and Wood 1984). Animal studies suggest that coronary blood flow increases out of proportion to the rise in cardiac output in normovolaemic anaemia (von Restorff et al. 1975; Biro 1982), but that the combination of severe acute anaemia with coronary stenosis results in myocardial ischaemia (Most et al. 1986; Biro et al. 1987; Levy et al. 1993). Studies of acute isovolaemic haemodilution confirm that reduction of circulating haemoglobin (Hb) concentration to 50 g/l in conscious healthy resting volunteers does not produce inadequate systemic O2 delivery as measured by decreased O2 consumption, increased lactate production or electrocardiographic changes (Leung et al. 2000); however, acute isovolaemic anaemia slows human reaction time, degrades memory, increases heart rate and decreases energy level to a significant if subtle degree (Weiskopf et al. 2002).
The implications for the restoration of blood volume in oligaemic patients are that: first, it is crucial to maintain the cardiac output by prompt restoration of the circulating blood volume; second, a moderate degree of anaemia should be well tolerated at least in previously healthy subjects provided that normovolaemia is maintained; and third, in patients whose coronary or cerebral arteries are diseased with limited ability to dilate and increase flow, or whose cardiac reserves are already depleted, any diminution in the O2 delivery may be damaging.
The Rate of Transfusion
As emphasized above, the primary aim in treating oligaemia is to maintain an adequate supply of O2 to vital organs. Provided that the cardiac output is maintained, some degree of anaemia can be tolerated. Although cardiac output can be maintained and even increased with anaemia, it is not maintained with oligaemia. If haemorrhage is moderate or severe, restoring the circulating volume immediately with a rapid infusion of crystalloid or colloid is preferable to delaying resuscitation until blood has been crossmatched. The added risks of uncrossmatched blood (‘universal donor’) are discussed elsewhere (Weiskopf 2010; see also Chapters 10 and 11).
When it is necessary to transfuse a unit of blood in a few minutes or less, rapid transfusion is safe as long as the patient is carefully monitored. Rapid rates of red cell transfusion cannot be attained by gravity infusion through a small cannula inserted in a peripheral vein. Methods of increasing the rate of flow are discussed below.
Technique of Rapid Transfusion
The Length and Internal Bore of the Cannula
Cannula length and size are important factors in limiting the rate of flow. The longer the catheter, the greater the resistance to flow and the less useful for rapid fluid infusions. Kestin (1987) measured the flow rates generated through all the intravenous cannulae available in the UK using a test prescribed by the British Standards Institution (BSI 1972). Distilled or deionized water was run under a pressure of 10 kPa (76 mmHg) from a constant level tank at 22°C via 110-cm tubing with an internal bore of 4 mm. The mean flow obtained through 14-SWG cannulae of all brands was 286 ± 35 ml/min, whereas through 16-, 18- and 20-SWG cannulae it was 162 ± 35, 91 ± 17 and 54 ± 14 ml/min respectively. However, the internal bore of the cannula and not necessarily the gauge (outside diameter) is the critical factor.
The Viscosity of the Blood
Blood is more viscous than water. Under test conditions at room temperature, the flow of blood (PCV = 0.45) through cannulae is only 50–60% of the flow of water (Farman and Powell 1969). When blood was infused at constant pressure through needles of various bore sizes, raising the Hb concentration from 12.6 to 14.3 g/dl slowed the flow rate by about 10% (Chaplin and Chang 1955). As blood temperature drops, blood viscosity increases. Warming blood with a PCV of 0.42 from 10°C to 36°C doubles the rate of flow (Knight 1968).
Venous Spasm
Infusing cold blood may cause local discomfort and venospasm, which will slow transfusion. The transfusion of warm blood or plasma may also induce venous spasm due to vasoconstrictor substances in plasma [see Mollison (1983), pp. 746–747]. In patients with peripheral circulatory failure due to oligaemia, the veins are constricted, so that the rate of flow of transfusion may be slower than is required. Local warming of the arm helps to relieve venous spasm, as does transdermal nitroglycerine applied as a patch distal to the infusion site (Wright et al. 1985). Despite the potential disadvantages, rapid transfusion of 1–2 units of blood taken directly from the refrigerator seems to be safe. Transfusion of larger amounts of very cold blood is undesirable (see Chapter 15).
Positive Pressure
Quadrupling the pressure gradient from a litre bag of 0.9% saline down an infusion set and cannula by enclosing the bag in a pressure cuff at 300 mmHg only doubles the flow rate through the cannula (Chen et al. 2002). The rather small increment in flow rate may be due both to the physics of flow through a tube and to the development of turbulence. When rapid transfusion is required, insertion of a large cannula is more efficacious than reliance on pressurizing the infusate through a smaller one. In practice, both pressurized infusion and large bore cannulae are used. Central venous cannulation with catheters of wide bore (for example, a pulmonary artery catheter of 8.5 gauge) is frequently performed in the management of severe haemorrhage, but a shorter 14-G peripheral catheter is usually more efficient. Pumps may be employed to transfuse or re-infuse blood at operations in which blood loss is massive, for example liver transplantation and major trauma. Multiple infusion sites are commonly used as well.
How Long to Continue Transfusion in Oligaemia
Transfusion with appropriate fluids should be continued until haemostasis has been achieved and clinical evidence of hypovolaemia has resolved.
A rise in systolic pressure to 100 mmHg is not an indication for stopping the transfusion. A relatively small transfusion given to an injured person may produce considerable clinical improvement without fully restoring blood volume. Evans and co-workers (1944) made serial estimations of plasma volume in injured subjects with peripheral signs of circulatory failure. They noted that after a small transfusion, for example 500 ml of blood, blood pressure might rise and the patient might appear much improved. Nevertheless, blood volume remained low for as long as 4 days. During the intervening period, the patient might easily lapse into shock again. The general practice is to restore normal blood volume if possible before taking a patient to operation. Most practitioners aim to return circulating volume to normal values if possible and if bleeding can be controlled. However, operation may be required at low circulatory volume if bleeding cannot be otherwise controlled. Global measures of organ perfusion such as base deficit and serum lactate may help guide fluid management during the first 24 h (Ivatury et al. 1996).
Failure to Respond to Transfusion
When an injured person’s blood pressure fails to respond to transfusion, two situations should be considered immediately. First, the patient may still be losing blood, so that transfusion does not succeed in restoring blood volume to an adequate level. Much later, infection may develop, so that the patient develops sepsis in addition to hypovolaemia. Operation should not be further delayed if the patient’s condition does not improve after transfusion of a reasonable volume, as surgery may help to deal with both underlying causes. A third concern when patients fail to respond to volume challenge is a cardiogenic cause; in this situation, if acute pericardial tamponade is not the issue, non-operative management may be the most prudent course.
Overloading of the Circulation
Although blood loss is commonly underestimated and circulatory overload of a patient who has lost blood through injury is unusual, volume overloading, characterized by engorgement of the neck veins and moist sounds on auscultation, does occur. However recent focus on causes of acute lung injury (ALI) and transfusion-associated acute lung injury (TRALI, Chapter 15) has led to the ‘rediscovery’ of transfusion-associated circulatory overload (TACO). In two retrospective multicentre analyses of orthopedic patients, the frequency of TACO ranged from 1–8%. (Popovsky et al. 1996; Bierbaum et al. 1999). As might be expected, advanced age is a risk factor and autologous blood is no safeguard, but surprisingly a setting of massive transfusion was not necessary; TACO was seen when only 1–2 units were transfused. The risk appears to be higher still (1/356 per unit transfused) in patients in intensive care settings (Rana et al. 1999). Haemovigilance studies confirm that TACO remains a significant cause of death in transfused patients. Overloading is best prevented by close attention to the state of the patient’s circulation. Frequent or continuous monitoring of central venous pressure provides the most reliable guide. The heart rate, arterial pressure, and core and peripheral temperatures can add helpful supplementary information about the degree of filling. Measurement of B-natriuretic peptide (BNP), a 32 amino-acid peptide, and a pro-peptide (NT-BNP), both secreted by ventricular myocardium in response to volume and pressure distention, can help in the diagnosis, but, like relying on evidence of hypoxemia, the results generally come a bit late to prevent the onset of TACO when blood is infused rapidly (Zhou et al. 2005; Tobian et al. 2008). These assays are not sufficiently specific to distinguish TACO from TRALI (Li et al. 2009).
Choice of Intravenous Fluid in Oligaemia: Use of Whole Blood
A long-sanctified fallacy maintains that no fluid is superior to whole blood in the treatment of haemorrhage, even with the proviso that blood, after storage in the cold for several weeks, contains membrane-modified, relatively inflexible, 2,3-DPG-depleted red cells and cellular detritus that may be temporarily less effective than fresher red cells in delivering and releasing O2 to the tissues (Klein 2003). No evidence supports this contention and its proponents appear to be dwindling. In any case, whole blood, and especially fresh whole blood – however that may be defined – is usually unavailable and rarely ordered even when it is.
Several factors limit the routine use of whole blood. From a public health perspective, blood is a scarce resource and should not be used when the major requirement is volume replacement and when an adequate substitute is available. From a patient care perspective, blood transfusion involves many hazards, of which the transmission of human immunodeficiency virus (HIV) is but one (Chapters 11, 15 and 16). Because a degree of anaemia can be tolerated by most patients, moderate haemorrhage can usually be managed effectively by the infusion of a crystalloid or colloid solution. If haemorrhage is severe enough to require the transfusion of red cells, red cell concentrates combined with crystalloid or artificial colloid solutions may be more appropriate than transfusion of whole blood. Infusion of red cell concentrates in combination with albumin fractions, a rich man’s reconstituted blood, has not been found superior to less expensive approaches to volume expansion (see below).
Whole blood still has its place in the treatment of massive haemorrhage. Whole blood from walking donors may be lifesaving for wounded soldiers at the battlefront when components are not available. A retrospective analysis of soldiers who sustained battlefield trauma and received fresh whole blood suggested that this component contributed to improved survival at 24 hours and 30 days (Spinella et al. 2009; Perkins et al. 2011). Many clinicians who treat rapidly bleeding patients agree with Schmidt (1978) that in hospital practice, a small but real need for whole blood exists. On the other hand, Lundsgaard-Hansen (1978) issued 80% of units as packed cells and supplemented these units with modified fluid gelatin when treating large haemorrhages. In the developing world, where often the capacity to produce components and store them safely is limited, whole blood may be life-saving and its use should not be discouraged.
The Need for Plasma Fractionation: the Use of Albumin
At present, plasma fractionation is driven by demand for two protein concentrates: albumin and immunoglobulin. Specific indications for the latter are well documented, whereas the need for albumin is a more controversial matter. Albumin is an effective plasma expander. An estimated 90–95% of infused albumin solution remains in the intravascular space (Payen et al. 1997). Normal colloid osmotic pressure is 28 mmHg and albumin accounts for 21.8 mmHg. Each gram of albumin attaches 18 g of water. However, fractionated human albumin is easily the most expensive volume expander available and several non-human-derived alternatives exist. Furthermore, the effect of albumin infusion varies significantly among patients and over time for any given patient (Schwartzkopff et al. 1980). Theoretical volume expansion of a 500-ml infusion of 4% albumin is approximately 400 ml. Double-label studies of haemodiluted surgical patients indicate that as haemodilution proceeds, the extravasation rate of albumin and fluid to the interstitial tissues increases, impeding the maintenance of isovolaemia (Payen et al. 1997). However, the effect is influenced by hydration status, endogenous albumin stores and accelerated albumin leakage into the extravascular space as a result of an underlying disease. In a prospective, randomized study of 475 patients admitted to an intensive care unit, albumin was found to offer no advantage over a gelatin colloid volume expander in terms of mortality, length of stay or incidence of pulmonary oedema or renal failure. Not surprisingly serum albumin levels were lower in the patients who received gelatin, although no clinical importance attaches to this observation (Stockwell et al. 1992a,b). A flawed meta-analysis has concluded that albumin administration may increase mortality in critically ill patients (Cochrane Injuries Group Albumin Reviewers 1998). However, this analysis included studies of albumin used for what might charitably be called uncommon indications as well as studies with small numbers of patients, so that the warning of possible increased risk when albumin is used as a volume expander may not be warranted. A more recent analysis of the Cochrane database that reviewed 57 trials and 3659 participants concluded that no single colloid solution is more effective or safe than any other (Bunn et al. 2003). A large randomized controlled trial in critically ill patients has confirmed the apparent equivalence of albumin and saline as resuscitation fluids (The SAFE Study Investigators 2004).
Infusions of Saline: Crystalloid or Colloid: What’s an Old Salt to Do?
Because delays associated with relying on the emergency provision of blood components may be harmful, resuscitation guidelines generally recommend early infusion of universally available crystalloid or colloid solutions. Debate has raged for more than 30 years as to whether crystalloid or colloid is preferable in the initial management of haemorrhagic shock (Farrugia 2011). The proponents of both views base their case on Starling’s equation, which states that the flow of fluid outwards through the walls of the capillaries (Jv) is proportional to the hydrostatic pressure (P) gradient between the capillaries and the interstitial space and inversely proportional to the protein oncotic pressure (π) gradient, namely:
where Pc is capillary hydrostatic pressure; Pt is interstitial fluid hydrostatic pressure; πc is plasma protein oncotic pressure; πt is interstitial fluid oncotic pressure; Kf is the filtration coefficient representing the permeability of the capillary membrane to fluids; and σ is the reflection coefficient representing the permeability of the capillary membrane to proteins.
The protein concentration of plasma is normally considerably higher than that of interstitial fluid. It seems logical to suppose that infusions of albumin or of other substances of equal or greater molecular weight would, given normal capillary permeability, remain in the intravascular space longer than would saline, which diffuses throughout the extracellular compartment. Based on an understanding of Starling’s law, colloid solutions have been developed and used for more than 70 years as volume expanders. Increasing osmotic pressure with colloidal products has remained an attractive theoretical premise for volume resuscitation. Colloids do increase osmotic pressure in clinical practice, but the effects are short-lived. Lower molecular weight colloids exert a large, if transient, initial osmotic effect, as the small molecules are rapidly cleared from the circulation. Larger molecules exert a smaller osmotic pressure, but one that is sustained longer. The main drawback to colloid therapy lies in pathological states with endothelial injury and capillary leak, precisely the clinical scenario in which colloids might be needed and are commonly given. The colloid solution may leak into the interstitium and exert an osmotic gradient, pulling additional water into the interstitium.
Four general types of colloid are available for clinical use. Albumin, the predominant plasma protein exerting some 80% of normal colloid osmotic pressure, remains the standard against which other colloids are compared (see Chapter 14). Dextrans of various molecular weights have been used to restore plasma volume and to prevent deep venous thrombosis in postoperative settings. Hydroxyethyl starch (HES), widely used as a plasma volume expander, provides equivalent plasma volume expansion to albumin, but has been shown to affect haemostasis and clotting assays. Although severe coagulopathies have been reported in sporadic cases, the newer HES formulations have not been shown to increase postoperative bleeding compared with albumin therapy, even in large doses (3 litres per day). Gelatins have been used extensively in some European countries, but have never been licensed in the US and have now been largely replaced by HES in other countries.
Measurements in rabbits have suggested that colloid solutions are more effective and persistent expanders of the plasma volume than is isotonic saline (Farrow 1967, 1977). In sheep that had about one-half of their blood volume removed, restoration of the left atrial pressure and cardiac output to baseline values with Ringer’s lactate required the infusion of two and a half times the volume of the blood withdrawn, whereas a second similarly bled group needed only to have the shed blood returned to achieve the same result. The authors could find no evidence of pulmonary capillary damage after this degree of haemorrhage (Demling et al. 1980). In a study of elderly patients resuscitated with either HES or Ringer’s lactate, together with packed red cells, much smaller volumes were required and a lower incidence of pulmonary oedema was observed in the group receiving colloid (Rackow et al. 1983). The pharmacodynamics of crystalloids and colloids have been studied in normal volunteers (Svensén and Hahn 1997). Despite the theoretical advantages compared with crystalloid therapy, these colloids have not been shown to decrease the risk of acute lung injury or to improve survival. The case for colloids has been summarized by Rady (1994).
An opposing case has also been presented. Several estimates of the protein concentration of pulmonary interstitial fluid have suggested that it is higher than in interstitial fluid elsewhere in the body (Staub 1974). In baboons that were subjected to haemorrhage and treated with either albumin or Ringer’s lactate plus their own shed blood, pulmonary oedema was more frequent when albumin was used, and albumin appeared to be extravasated into the pulmonary tissue (Holcroft and Trunkey 1974; Lewis et al. 1979).
Numerous clinical studies support these animal findings. Virgilio and co-workers (1979) compared the results of infusing albumin and Ringer’s lactate, both with packed red cells, in two groups of patients undergoing major vascular surgery. To achieve a predetermined circulatory state and urine output, the patients who were given albumin required the infusion of smaller volumes than did those who were given crystalloid, but showed a higher incidence of pulmonary oedema. Moss and co-workers (1981) studied a series of young injured patients who were given washed red cells and Ringer’s lactate, supplemented in one-half of the cases with albumin. No patient developed pulmonary oedema and the total volume of Ringer’s lactate required to correct hypovolaemia was not reduced by the addition of protein (Pearl et al. 1988).
Clinical and experimental studies support the concept that haemorrhagic shock leads to a cellular insult that promotes interstitial fluid expansion at the expense of the plasma volume after successful control of haemorrhage. This expansion of interstitial fluid is associated with plasma protein reduction; the degree of hypoproteinaemia is directly related to the shock insult as measured by a systolic pressure below 80 mmHg and by the number of transfusions required during phase I. During the interstitial fluid expansion period, the percentage of protein exiting the plasma volume is below normal so that the interstitial fluid protein accumulates because even less protein returns to the plasma space. During the subsequent mobilization phase, the plasma proteins increase progressively as the interstitial fluid space contracts. This increase in the plasma proteins occurs when the percentage of extravascular albumin flux is actually increased so that the mechanism for increased plasma proteins must reflect an even greater increase in the protein flux out of the interstitial fluid space into the plasma. The supplementation of the resuscitation regimen with any colloid forces an even greater amount of colloid flux from the plasma into the interstitial fluid during the fluid sequestration phase and prolongs the re-entry of proteins from the interstitial fluid space into the plasma during the subsequent mobilization phase. This phenomenon is associated with multiple adverse effects on organ function and coagulation and an increase in the mortality rate (Lucas and Ledgerwood 2003).
In the face of such conflicting evidence, conclusions are difficult to draw. The human studies were conducted on widely differing groups of patients, often involved different measurements, and all had different endpoints. In a few instances, they seem designed to reinforce the preconceived ideas of the workers concerned. The results of animal studies are also at variance, and, illuminating though such studies may be, are not necessarily applicable to human subjects. A landmark randomized study of resuscitation involving nearly 7000 critically ill patients found that 4% albumin and normal saline were associated with equivalent overall mortality in a heterogeneous population of patients in the intensive care unit (The SAFE Study Investigators 2004). Analyses of most subgroups revealed no significant differences between patients who received saline and those who received albumin, but in the 1126 trauma patients, albumin was associated with a trend towards increased mortality, and among the 1218 patients with severe sepsis, albumin was associated with a trend towards reduced mortality. Both findings merit further study. One subgroup may prove the exception. In a post hoc study of critically ill patients with traumatic brain injury, fluid resuscitation with albumin was associated with higher mortality rates than was resuscitation with saline.
The subgroup of patients with Glasgow Coma Scale (GCS) scores of 3 to 8 constituted 160 (69.3%) of the albumin group and 158 (69.0%) of the saline group. Demographic characteristics and indexes of severity of brain injury were similar at baseline. At 24 months, 71 of 214 patients in the albumin group had died, as compared with 42 of 206 in the saline group, a highly significant difference. Among patients with severe brain injury, 61 of 146 patients in the albumin group (41.8%) died, as compared with 32 of 144 in the saline group, again highly significant, whereas among patients with Glasgow Coma Scores of 9 to 12, death occurred in 8 of 50 patients in the albumin group (16.0%) and 8 of 37 in the saline group (21.6%).
It seems reasonable to conclude that in previously fit young patients, with the possible exception of those with traumatic brain injury, timely restoration of the blood volume is more important than how the restoration is accomplished, whether with a colloid or a crystalloid solution. In a Cochrane analysis of 63 eligible randomized clinical trials involving different patient groups, crystalloids and colloid solutions, there was no evidence that resuscitation with colloids reduced mortality, compared to resuscitation with crystalloids, in patients with trauma, burns or following surgery (Perel and Roberts 2007). As colloids are not associated with an improvement in survival, and as they are more expensive than crystalloids, it is hard to see how their continued use in these patients can be justified outside the context of trials. Clinical judgment, convenience and cost should dictate the choice of solution infused. Most experts agree that when crystalloid solutions are used, the volume infused should equal at least three times the estimated volume of shed blood, although dilution kinetics depend on the specific solutions used (Drobin and Hahn 2002; Svensen et al. 2009). In the SAFE study, the ratio of the volume of albumin to the volume of saline administered over the first 4 days was only 1 : 1.4 (The SAFE Study Investigators 2004). In the elderly or in patients with poor renal or cardiac function, it may be prudent to avoid massive infusions of saline as well as rapid infusions of a highly concentrated (25%; 12.5 g of albumin in 50 ml of buffered saline with an oncotic pressure of 100 mmHg) albumin solution that might lead to cardiac decompensation (Roberts and Bratton 1998). Characteristics of commonly used crystalloid and colloid solutions are found in Table 2.1.
Table 2.1 Characteristics of selected resuscitation fluid.
Source: Adapted from Committee on Fluid Resuscitation for Combat Casualties (1999). Pope 1999. Reproduced with permission of the National Academy of Sciences, Courtesy of the National Academies Press, Washington, D.C.
The Advanced Trauma Life Support (ATLS) Programme for Physicians, initiated in the US and adopted by at least 15 other countries, directs attention to the management of patients in the first ‘golden’ hour after injury when a high proportion of deaths occurs and when proper care might be expected to improve outcome. The ATLS protocols on hypovolaemia include guidelines on the clinical assessment of the degree of haemorrhage and advocate aggressive fluid replacement with crystalloid (2000 ml for an adult, 20 ml/kg for children), supplemented by red cells, if necessary, infused through two large-bore (14-G) cannulae placed peripherally (American College of Surgeons Committee on Trauma 1997). For uncontrolled bleeding and massive haemorrhage, the paradigm is changing to one of minimal volume replacement (so not to disturb clot formation) and damage control surgery.
Infusions of Hypertonic Saline: More Salt in the Wounds
Infusions of small volumes (about 4 ml/kg body weight) of hypertonic solutions (7.2–7.5% sodium chloride) over a short period (2 min) have been used in the initial resuscitation of both patients and experimental animals with hypovolaemic shock since 1980 (Kramer et al. 1986; Holcroft et al. 1987). Dramatic instantaneous improvements in cardiac output, arterial pressure, acid–base balance and urine flow, persisting for a few hours, have been observed. Hypertonic solutions produce their beneficial effects by expanding the circulating volume at the expense of the intracellular volume. Several studies have shown that the efficiency of hypertonic saline may be enhanced by the addition of dextran (Wade et al. 2003). Hypertonic saline solutions, with or without supplementary colloid, appear to have a place in the immediate management of an injured patient; an infusion may be started at the site of the accident before the patient is removed to hospital (see Messmer 1988). An early study found a survival advantage for patients who required surgery, and the hypertonic saline dextran group appeared to have fewer complications (Mattox et al. 1991). Multicentre trials, however, comparing lactated Ringer’s solution and hypertonic saline with dextran failed to show convincing overall differences in survival. The advantages of hypertonic saline resuscitation include its haemodynamic effects and its effects on lowering intracranial pressure in brain-injured patients. However, in the largest randomized control trial of its kind, the US National Heart Lung and Blood Institute halted its trial of hypertonic saline in traumatic brain injury after review of 1000 patients indicated that the treatment was no better than the normal saline control (Bulger et al. 2010). A parallel trial in trauma patients with haemorrhagic shock was halted when hypertonic saline failed to improve patient survival. Although multiple studies have suggested further benefits in modulating the inflammatory response that occurs during shock, this benefits could not be translated into clinical benefit. A meta-analysis in the Cochrane database concludes from an analysis of 12 studies of patients with trauma burns or surgery that insufficient data exist to recommend between hypertonic and isotonic saline (Bunn et al. 2002; Kwan et al. 2003).
Plasma Alternatives (Substitutes)
The immediate effect of blood transfusion in haemorrhage derives mainly from the sustained increase in blood volume that it produces. Bayliss (1919) showed that a fluid that contained colloids with an osmotic pressure similar to that of plasma proteins could be used as a substitute for blood. Plasma is a complex solution containing numerous lipids, proteins, hormones, electrolytes and functions; solutions designed to restore or sustain blood volume should probably be referred to as ‘plasma alternatives,’ but as the name ‘plasma substitute’ has gained widespread acceptance, the terms will be used interchangeably.
Numerous substances have been tried as plasma substitutes but not one of these has entirely fulfilled the theoretical requirements. For example, gum arabic, introduced by Bayliss, fell into disrepute because it was found to be stored in the tissues. Human serum albumin has been widely used as a plasma substitute since the Second World War. Characteristics of albumin plasma protein fractions are discussed in Chapter 14.
The main qualities required of a plasma substitute are: (1) retention in the circulation for relatively long periods after infusion; (2) minimal risk of allergic reactions; (3) freedom from unwanted side-effects, including coagulopathy and transmission of infection; (4) complete metabolism after clearance from the circulation; (5) immunological compatibility; (6) long shelf-life under practical storage conditions; and (7) reasonable cost. No product provides all of these features.
All artificial colloids, unlike albumin, are polydisperse, composed of mixtures of molecules of widely varying size and molecular weight. Ideally, a preparation should contain no molecules of molecular weight below 70 kDa, as these will be rapidly excreted by the kidney. On the other hand, to ensure that only a small proportion of such molecules are present, it may be necessary to accept some molecules with a molecular weight greater than 250 kDa. The low molecular weight starch Voluven still has an in vivo mean molecular weight of 70–80 kDa following infusion and thus exceeding the renal threshold.
Size Matters
In describing the average molecular weight of preparations that contain molecules of widely varying size, it makes a substantial difference whether one gives the number average (mn) or weight average (mw).
(mn) is obtained by dividing the total weight of all molecules present by the total number of molecules. (mw) is obtained by summing the products of the weights of all molecules of a particular size multiplied by their molecular weight and dividing the sum by the total weight of all the molecules. For mixtures of different molecular weight, (mw) is always greater than (mn) and the ratio (mw)/(mn) gives a good measure of the degree of scatter of molecular weights in the mixture. Values of mw and (mn) for dextran and gelatin preparations provided in this chapter are either taken from the monograph by Gruber (1969) or have been obtained from manufacturers.
Clinical Dextran
Dextrans are polydisperse linear D-glucose polymers produced by bacteria grown on sucrose-containing media. Commercial dextran preparations can be stored at room temperature. Dextrans are not used widely in the US where they have been generally supplanted by starch and albumin solutions.
Two preparations of clinical dextran are commonly used, dextran 70 (mw 70 kDa, mn 39 kDa) and dextran 40 (mw 40 kDa, mn 25 kDa). The effects on the plasma volume of rabbits of these two dextrans, and also of dextran 110, modified fluid gelatin and a ‘plasma protein (albumin) preparation’, were compared by Farrow (1967, 1977). The main conclusions were as follows: the expansion of plasma volume depends mainly upon the weight of dextran in the circulation and is not directly related to the molecular weight of the preparation. However, in terms of maintenance of expanded plasma volume, the average molecular weight of the infused dextran is very important because of the rapid excretion of small molecules. Dextran 40 is supplied as a 10% solution and after infusion, is filtered and excreted unchanged in the urine; other dextran preparations are supplied as 6% solutions, so that for equivalent volumes the immediate effect of dextran 40 is considerably greater; the infusion of a volume equivalent to 22% of the rabbit’s plasma volume produces an immediate increase of about 35%. Nevertheless, the effect is maintained for only a few hours. Higher molecular weight dextrans tend to aggregate red cells in vitro, which can cause some confusion during compatibility testing (see below). Compared with dextran 110, dextran 70 has the advantage of producing negligible red cell rouleaux formation, but the duration of plasma volume expansion is sacrificed and the material remains effective for only about 24 h, whereas at the end of this period dextran 110 continues to produce some increase in plasma volume. The larger dextrans undergo gastrointestinal clearance or cleavage within the reticuloendothelial system by dextranases. Plasma protein fraction produces a much smaller increase in plasma volume than an equivalent volume of 6% dextran, but the effect is well maintained. Dextran 40 as a plasma volume expander is relatively contraindicated in the treatment of shock, because it may obstruct the renal tubules (Rush 1974). Similarly, the infusion of large amounts of dextran 40 in patients who already have slight impairment of renal function may precipitate acute renal failure; six cases were reported by Feest (1976), who advised that not more than 1 litre per day should be administered and that if urinary output falls, the infusions should be stopped and the patient treated with diuretics and a high fluid intake.
A 3% solution of dextran 60 (molecular weight 60 kDa) has been used in Europe. Despite its low concentration of dextran, dextran 60 seems to be an effective plasma substitute. For example, in a small, randomized study of patients undergoing aortic surgery, and receiving fluid replacement of red cells with either Ringer’s lactate or 3% dextran 60, almost three times the volume of crystalloid was needed to achieve adequate circulatory filling with Ringer’s lactate in comparison with the dextran solution (Dawidson et al. 1991).
Allergic Reactions to Dextran
Many types of clinical dextran are capable of acting as antigens in humans. Moreover, anti-dextrans may be found in the serum of individuals who have never received dextran infusions (Kabat and Berg 1953). The sera of elderly people and of patients with inflammatory disease of the intestinal tract may contain large quantities of dextran and reactive antibodies of the IgG class, suggesting that dextran may be ingested in food or produced by microorganisms in the gut (Palosuo and Milgrom 1980).
Severe reactions to dextran infusions are confined to individuals who have high titres of IgG anti-dextran before they receive any dextran parenterally (Hedin and Richter 1982). The clinical manifestations result from the formation of immune complexes between the dextran infused and the circulating antibodies. (Hedin et al. 1979) The inhibition of immune complex formation by a hapten (dextran 1) was formulated to prevent severe dextran-induced anaphylactic reactions (Messmer et al. 1980). Although the routine use of dextran 1 immediately before the infusion of dextran 70 or dextran 40 has been associated with a reduction in the reported incidence of severe reactions in Sweden (from 22 per 100 000 units between 1975 and 1979 to 1.2 per 100 000 units between 1983 and 1985) (Ljungstrom et al. 1988), cases continue to be reported (Berg et al. 1991).
Unwanted Side-Effects of Dextrans
Abnormal Bleeding
Dextran may interfere with haemostasis, apparently by affecting platelet function. A fall in factor VIII levels was observed when as little as 500 ml of 6% dextran was given to 16 volunteers; no such change occurred in three others who were given normal saline (Aberg et al. 1979). These observations have led many authorities to advise that the total dosage of dextran administered should not exceed 1.5 g/kg, or about 1.5 litres of 6% solution for a 70-kg patient. However, some clinicians have given twice this amount to patients who are undergoing elective orthopaedic surgery, with no apparent ill effects. The platelet counts were lowered in these patients but factor VIII levels were unchanged (Bergman et al. 1990). Dextran has been reported to have fibrinolytic properties that may contribute to its anticoagulant effects (Eriksson and Saldren 1995).
Interference with Crossmatching
The tendency of dextran to cause rouleaux formation increases with molecular weight; with a molecular weight of 60 kDa, a concentration of 2% is needed, but with a molecular weight of 270 kDa, only 0.4% (Thorsen and Hint 1950). Despite the wide range of molecular weights in dextran preparations, the infusion of presently available preparations does not lead to rouleaux formation in tests with untreated red cells. On the other hand, if enzyme-treated cells are used, even infusions of dextran 40 can lead to intense aggregation. When the possibility exists that large amounts of dextran will be administered, blood should be taken for crossmatching before the infusion is begun.
Gelatin
Gelatins are prepared from bovine collagen treated with alkali and suspended in aqueous solution. Preparations of a molecular size large enough to be well retained in the circulation do not remain fluid at low temperatures, a serious disadvantage in a preparation that is essentially needed for emergency conditions. The T1/2 of injected modified fluid gelatin, such as that of dextran 40, was found to be 2–3 h, comparable to that of Voluven and substantially shorter than the 6 h T1/2 of dextran 60 and HES (Ring et al. 1980).
Two types of gelatins that remain fluid under normal ambient temperatures are available (Saddler and Horsey 1987). In all gelatin preparations, the range of molecular weights is wide; about 70% of molecules are below the renal threshold.
Gelatin-induced volume expansion lasts about 3 h in healthy subjects but may be reduced in patients with sepsis (Salmon and Mythen 1993). The urea-linked gelatins contain high concentrations of calcium, so they must be used cautiously when infused with citrated blood components or clotting may occur. As mentioned below, allergic reactions seem to be somewhat more common after gelatin than after other plasma substitutes.
Perhaps the greatest advantage of modified fluid gelatin over other plasma substitutes is its apparent lesser tendency to contribute to bleeding. Compared with dextran and most HES preparations, gelatins have only a slight effect on platelets; whereas both dextran and HES lengthen the partial thromboplastin time, gelatin has no effect (Harke et al. 1976). In experiments on irradiated thrombocytopenic dogs that were subjected to rapid haemorrhage of 25 ml of blood per kilogram, replaced either by oncotically equivalent volumes of 15 ml/kg of 6% dextran or 4% modified fluid gelatin, the output of red cells into the thoracic duct lymph was three times greater in three out of six dogs treated with dextran, but was no greater than before bleeding in the dogs treated with gelatin (Lundsgaard-Hansen 1978). Furthermore, in a controlled prospective study of patients undergoing elective surgery, in those patients receiving 1000 ml of 6% dextran 70, bleeding times postoperatively were 50 s greater than preoperatively, whereas in patients receiving 1500 ml of 4% modified fluid gelatin, the increase was insignificant (3.8 s). Three patients survived after receiving more than 10 litres of modified fluid gelatin within 24 h (Tschirren et al. 1973). Sensitive in vitro assays can detect haemostatic defects that result from haemodilution alone (Casutt et al. 2010).
Hydroxyethyl Starch
Hydroxyethyl starch (HES) is a modified natural polymer that has been used frequently for plasma volume expansion in trauma and surgery. Because native starches are rapidly hydrolysed by [α-]amylase, commercially available HES preparations are extremely polydisperse polymers of natural amylopectin, chemically modified by hydroxyethylation at the glucose subunit carbon atoms (C2, C3 or C6). The molar substitution (moles of hydroxyethyl residues per mole of glucose subunits), the pattern of hydroxyethylation (C2/C6 ratio) and the in vivo mean molecular weight (mw) are important characteristics that affect the in vivo function of different preparations. The higher the molar substitution and C2/C6 ratio, the slower the starch is metabolized. mw is less important. The physicochemical characteristics of HES solutions are related to different safety profiles of various HES types with respect to coagulation and renal function. In particular, after the repeated administration of certain HES solutions with high MS and, to a lesser extent, a higher mean mw, large molecules may accumulate in plasma because of decelerated enzymatic degradation. Macromolecules that accumulate in plasma are known to decrease plasma concentrations of coagulation factor VIII, von Willebrand factor (VWF) and ristocetin cofactor, although the mechanism responsible for the adverse effect on factor VIII/VWF complex is not fully understood (Treib et al. 1999). Some of the older, less readily metabolized HES products reportedly lead to deterioration of renal function. HES deposits in macrophages and has been associated with persistent, often severe pruritus, although the frequency of this side effect is unknown (Bork, 2005).
In the US, HES is supplied as a 6% solution in 0.9% saline (mw 450 kDa, mn 65 kDa), a 6% HES 130/0.4 in 0.9% saline, a 5% solution, and 6% HES in balanced electrolyte solution. A variety of other formulations are available in Europe and other countries (Dieterich 2003). As far as initial retention in the circulation is concerned, HES appears to be similar to dextran 70 [Gruber (1969), p. 140], although small amounts of HES tend to persist in the circulation for long periods (see below).
HES is infrequently associated with allergic reactions. In one study, only eight untoward reactions were observed in just over 10 000 recipients; only one of the reactions was of a severe anaphylactic type (see below).
Like dextran, most HES preparations interfere with blood coagulation, although to a lesser extent (Karlson et al. 1967). Coagulopathy resulting from HES administration is well documented for high-molecular-weight, highly substituted HES solutions (Treib et al. 1996). Particular concern has been expressed about reports of increased bleeding in patients who undergo open-heart surgery, especially as cardiopulmonary bypass independently induces a decline in platelet von Willebrand receptor glycoprotein IB. Some centres add 6% HES to the pump prime solution. In a review of 444 patients with coronary artery bypass graft (CABG), blood loss at 4 and 24 h was significantly increased in the 234 patients who received intraoperative HES (Knutson et al. 2000). A meta-analysis that included 653 patients arrived at the same conclusion (Wilkes et al. 2001).
A variety of other HES solutions that differ greatly in their pharmacological properties exist worldwide. Slowly degradable high-molecular-weight HES 450/0.7 and medium-molecular-weight HES 200/0.62 have a high in vivo molecular weight and are eliminated slowly via the kidneys. As a result, these starches have a relatively long-lasting volume effect. When higher volumes (>1500 ml) are infused, large molecules accumulate in the plasma. This can result in bleeding complications due to decreased factor VIII/von Willebrand factor, platelet function defects, incorporation into fibrin clots and an unfavourable effect on rheological parameters. Rapidly degradable medium-molecular-weight HES 200/0.5 or low-molecular-weight HES 70/0.5 is quickly split in vivo into smaller, more favourable molecule sizes, resulting in faster renal elimination, shorter volume effect and fewer adverse effects on coagulation and rheological parameters. HES 130/0.4 was especially designed to minimize influence on coagulation (Franz et al. 2001). In clinical trials the effects on coagulation parameters (von Willebrand factor, factor VIII, ristocetin cofactor) were found to be substantially lower than those of pentastarch (Kozek-Langenecker 2005). Based on the trial data and meta-analysis of seven trials, one can say that the effectiveness of HES 130/0.4 is at least comparable to that of other HES solutions, and the effect on blood loss appears to be diminished (Gandhi et al. 2007; Kozek-Langenecker et al. 2008).
HES is commonly used to increase the yield of granulocytes from donors giving blood via cell separators (see Chapter 17).
Comparisons Between Plasma Substitutes
Comparisons may be made on the grounds of retention in the circulation, incidence of allergic reactions, unwanted side-effects, such as interference with platelet function, catabolism and excretion from the body, and cost. Dextran 70 and HES appear to be retained about equally well, but gelatin preparations, with smaller molecular weights, are excreted more rapidly.
All plasma substitutes have been associated with allergic reactions. In a prospective survey, reported in 1977, the incidence of reactions of all kinds, from skin symptoms alone at one extreme, to cardiac arrest at the other, was 0.69% for dextran 60/75; 0.007% for dextran 40; 0.085% for HES; 0.115% for gelatin; and 0.014% for plasma protein solutions (either whole serum or albumin). About one-quarter of the reactions were life-threatening; the highest incidence was found with gelatin solutions (0.039%), the next with dextran 60/75 (0.017%), then HES (0.006%) followed by plasma protein solutions (0.004%), with dextran 40 last with 0.002% (Ring and Messmer 1977). Gelatin is less likely than the other two plasma substitutes to interfere with platelet function (as is HES molecular weight 130 000) and thus less likely to provoke bleeding. Gelatin is rapidly catabolized, and dextran is also completely broken down, but some doubts remain about HES, which tends to persist for long periods in the circulation. In volunteers infused with 7 ml/kg of HES or dextran 60, after 17 weeks the plasma HES concentration was still 1% of the post-infusion level, whereas after 4 weeks, dextran was undetectable (Boon et al. 1977).
Red Cell Substitutes
Synthetic and Hb-based O2 carriers (blood substitutes, red cell substitutes, O2 therapeutics) are discussed in Chapter 18.
Infusion Solutions: Some Final Thoughts
The emphasis in this chapter has been on fluid resuscitation for trauma and massive haemorrhage. However intravenous solutions are used ubiquitously, have differing constituents, acid–base characteristics, side effects, and indications for specific patient settings. Like other medications, intravenous solutions should be titrated to physiologic endpoints (Kaplan and Kellum 2010). It is particularly unwise to mix fluids other than normal saline with blood components lest haemolysis, agglutination or clotting ensue.
Transfusion in Special Clinical Settings
Massive Transfusion
Transfusion is conventionally regarded as ‘massive’ when a volume equivalent to the patient’s normal blood volume is given within 24 h. This definition is clearly arbitrary. Transfusion can also be considered massive when >50% of blood volume (>5 u stored RBC) is replaced in 5 hours or blood loss exceeds 150 ml/min. All of these situations may be life-threatening. The complications of this syndrome, bleeding, hypothermia, acidosis and a variety of electrolyte derangements, may occur when one-half of this volume is given over a few hours or when several blood volumes are replaced over a period of days (Rutledge et al. 1986).
The clinical problems that arise in massively transfused patients are due first to the condition that caused the haemorrhage, and second to the effect of replacing normal whole blood with stored citrated blood, possibly supplemented by plasma, platelets, and plasma expanders. Coagulopathy, hypothermia, and acidosis form the ‘lethal triad’ of massive transfusion. Although a detailed consideration of clinical conditions associated with haemorrhage is beyond the scope of this book, some of these conditions, for example disseminated intravascular coagulation and sepsis, produce effects that may be attributed to the massive transfusion. Some of the consequences of replacing large volumes of the patient’s blood with similar volumes of stored citrated blood are as follows:
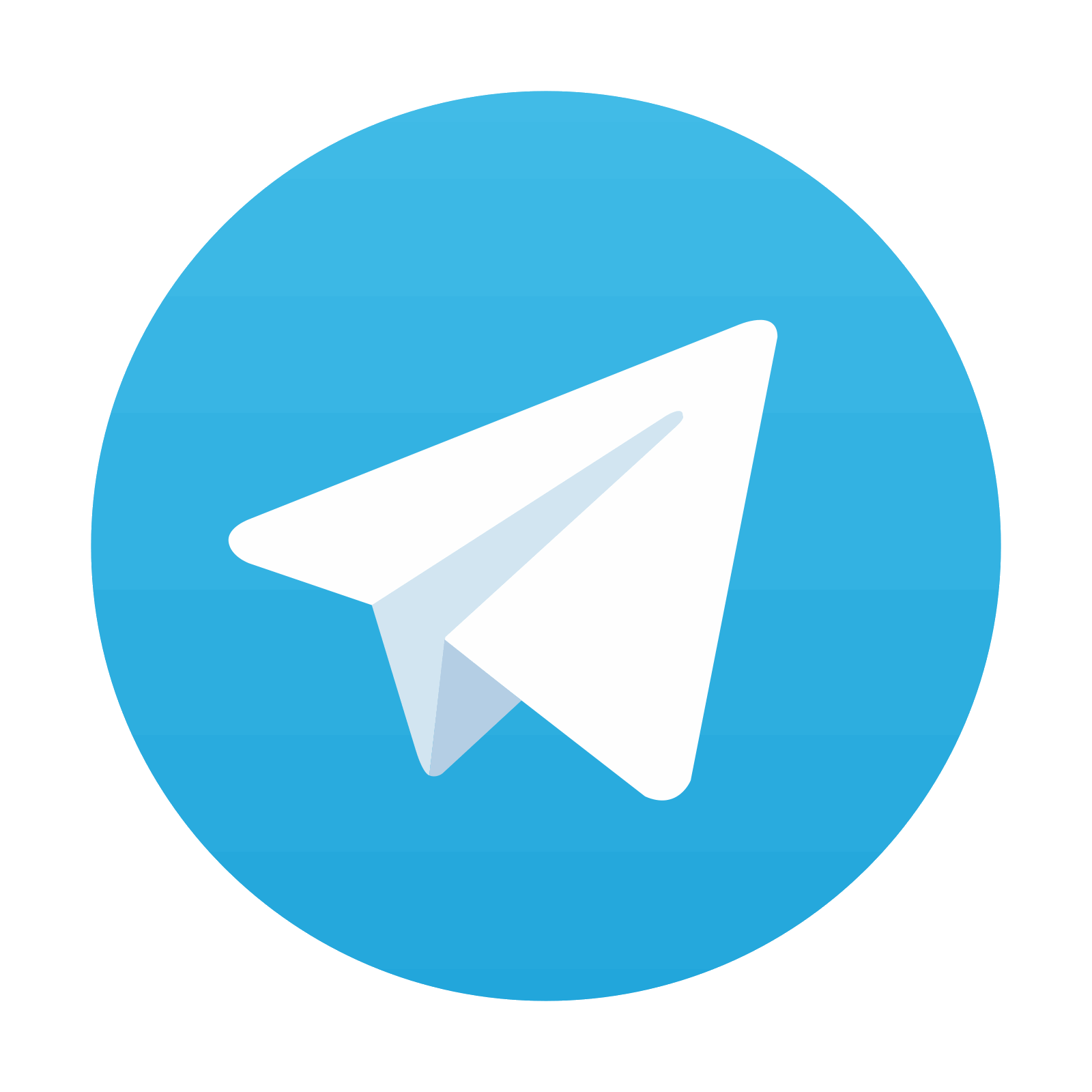
Stay updated, free articles. Join our Telegram channel
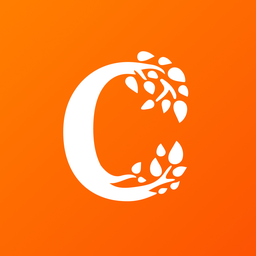
Full access? Get Clinical Tree
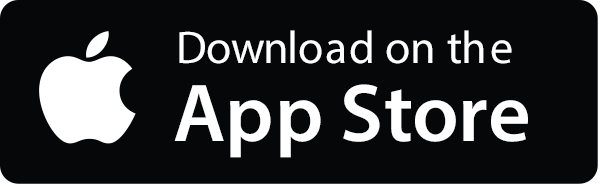
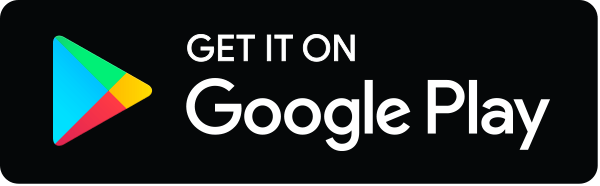