Fig. 10.1
A general classification of tumor immunotherapeutic approaches
To target and destroy cancer, the immune system must be able to recognize a given cancer antigen and label it as a “foreign invader” [1]. Tumor antigens (TAs) can be roughly divided into two different classes according to the specificity of antigen: tumor-associated antigens (TAAs) and tumor-specific antigens (TSAs). TAAs are expressed in some normal tissues at low levels but overexpressed in tumor cells. In comparison, TSAs are expressed exclusively on tumor cells, including (1) cancer-testis antigens (CTAs), which is shared across multiple tumor types, but is typically restricted to non-MHC-bearing germ cells such as those found in adult testis tissue; (2) viral antigen, which is encoded by viral oncogenes and restrictively expressed in virus-associated tumors; and (3) neoantigen, the immunogenic product of genetic mutation in tumor cells, which can be processed, be presented to T cells, and activate antitumor immune responses.
With this in mind, the following section is focused on the topic of tumor vaccines and its application to gastric cancer immunology. It seeks to outline the theoretical basis, historical trajectory, present-day status, and potential future applications for vaccine-based immunotherapy.
10.2 Scientific Basis of Immunotherapeutic Tumor Vaccines
10.2.1 MHC Molecules and T-Cell Epitopes
A MHC class I molecule consists of a polymorphic heavy chain, a β2-microglobulin subunit, and a peptide anchored to the binding groove that is located in the heavy chain. Classical MHC class I molecules include HLA-A, HLA-B, and HLA-C, which are encoded by genes at the HLA-A, HLA-B, and HLA-C loci, respectively. There are also some nonclassical MHC molecules with immunomodulatory functions, such as HLA-E, HLA-F, and HLA-G. Several hundred human heavy chain alleles have been discovered (http://www.allelefrequencies.net/). The peptide ligands binding to MHC class I molecules are generally composed of 9–10 amino acids. MHC class I peptides are predominantly derived from endogenous degraded proteins. This process begins with cleavage of the endogenous proteins by canonical proteasome and immunoproteasome with different cleavage preferences. Cleaved peptides in the cytosol are translocated into the endoplasmic reticulum and assembled into MHC class I molecules. Finally, the assembled MHC class I molecules are translocated to the cell membrane and recognized by T-cell receptors on T lymphocytes. Exogenous antigens can also generate MHC class I peptides through cross-presentation pathways. In this case, exogenous proteins are endocytosed into professional antigen-presenting cells (APCs) and then processed and loaded onto MHC class I molecules. MHC class II molecules are comprised of ligands with 12–26 amino acids. MHC class II peptides are mainly produced by professional APCs, such as DCs, macrophages, and B cells. Exogenous proteins are internalized, proteolyzed, and assembled into MHC class II molecules in the endosomes and lysosomes of the professional APCs. MHC class II molecules can also bind peptides originating from endogenous proteins by other processing pathways.
10.2.2 Identification of Tumor Antigens and Their Epitopes
10.2.2.1 Identification of Tumor Antigens
Different methods are developed to identify tumor antigens. Tumor-reactive T cells from cancer patients and tumor cell lines were used in the classical strategy for identifying T-cell antigens and their epitopes. Briefly, the tumor cDNA library is transfected into target cells expressing the appropriate HLA molecules. T cells deriving from tumor-infiltrating lymphocytes (TILs) or the peripheral blood mononuclear cells (PBMCs) are then adopted to identify T-cell antigens. The CTL epitope is subsequently defined through cDNA truncation and peptide recognition. Many T-cell antigens and their epitopes—including MAGE families, MART-1, tyrosinase, and gp100—are defined using this strategy. This direct immunological strategy is still the major strategy for identifying tumor-specific T-cell antigens and epitopes.
Gene-expression profiling is an approach used to identify overexpressed antigens found in tumors. This is because some of the genes overexpressed in tumor cells are involved in tumor growth and metastasis. The corresponding proteins of these overexpressed genes have been postulated to be useful tumor antigens. For example, the telomerase catalytic subunit is overexpressed in tumor cells and can be recognized by cytotoxic T lymphocytes [4].
Another method is serological screening of recombinant cDNA expression libraries (SEREX) and used to analyze B-cell responses in cancer patients in order to identify T-cell targets. NY-ESO-1, tyrosinase, and MAGE are examples of tumor antigens that have been identified via the SEREX strategy.
10.2.2.2 Identifying T-Cell Epitopes
Two strategies are currently being used to define T-cell epitopes and are based on different starting points. One is used in the case of knowing a T-cell reaction against tumor cells, without information regarding its specificity. Another begins with known tumor antigens, with allele-specific peptides being predicted and validated via a reverse immunological approach.
- 1.
Identification of CTL epitopes via T-cell recognition
The classical approach for T-cell epitope identification is to investigate the specificity of a tumor-specific CTL clone in order to define its epitope. Tumor-specific CTL clones from the patients are adopted to screen a tumor cDNA library. The minimal epitope sequences are then revealed by truncation experiments. An alternative approach is to use MHC molecules presenting the relevant peptides. These molecules are then purified using biochemical methods. The mixture of MHC ligands is eluted from the MHC molecules and fractionated using high-performance liquid chromatography (HPLC). Finally, the fractions recognized by T cells are then analyzed using tandem mass spectrometry (MS/MS)-mediated sequencing.
- 2.
Identification of CTL epitopes starting with tumor antigens
In comparison, this approach begins with known tumor antigens rather than preexisting T cells. Epitope prediction is the first and most important step to identifying CTL epitopes. Many software and online programs (e.g., BIMAS24, SYFPEITHI, and NetMHC) have been developed to predict peptides that have the possibility to bind with HLA molecules. In addition, other prediction methods can be adopted in order to more accurately predict the epitope. These strategies include proteasomal cleavage site and TAP translocation prediction methods. Once possible epitopes have been selected, they should be validated in order to demonstrate the presentation and immunogenicity of the predicted epitope. Peptide-specific T cells induced from human PBMC or HLA class I transgenic mice are often used as tools to test the natural presentation and immunogenicity of the epitope.
10.3 TAA-Based Tumor Vaccines
10.3.1 Peptides Derived from TAs and Their Properties
Tumor antigens (TAs) are the molecules specifically expressed or overexpressed by tumor cells. There are two types of tumor antigens, defined according to their tumor specificity [5]. Antigens with high tumor specificity include viral antigens, neoantigens, and cancer-testis antigens. Differentiated and/or overexpressed antigens belong to the class of antigens with low tumor specificity.
Some tumors are accompanied by viral infection. For instance, Epstein-Barr virus (EBV) was the first human virus to be directly associated with a wide range of cancers, including Burkitt’s lymphoma, Hodgkin’s disease, nasopharyngeal carcinoma, and gastric cancer [6]. Moreover, almost all cervical cancer cases (95%) are caused by the human papilloma virus (HPV) [7]. Finally, about 80% of hepatocellular carcinoma (HCC) is due to hepatitis B virus (HBV) or hepatitis C virus (HCV) infections [8]. Importantly, the connection between viral infection and cancer signifies that the virus antigens expressed by infected cells provides a source of distinctive tumor antigens.
Neoantigens are tumor antigens encoded by the mutated genes of tumor cells. They are promising immunogens and immunotherapeutic targets due to their tumor specificity as well as the lack of central tolerance [9]. Along with the development of whole-genome sequencing and the strategies used to systematically identify tumor neoepitopes, personalized tumor vaccines derived from neoantigens have been designed and utilized in both basic and clinical studies.
Cancer test antigens are proteins normally expressed in germline and trophoblastic cells, but are abnormally expressed in various human tumors (e.g., families of MAGE, BAGE, NY-ESO1, and SSX). They are immunogenic and highly tumor specific, so vaccines derived from them have been studied as safe and therapeutically beneficial.
Differentiated antigens are derived from proteins that are expressed in malignant and normal cells with the same lineage. Most of the discovered, differentiated antigens are expressed in melanoma cells. Moreover, melanoma differentiated antigens (MDAs) can be detected in melanoma cells as well as normal melanocytes. Antigen-specific T cells that have a high affinity to MHC complexes and that are loaded with MDA-derived peptides have been identified from the blood and tumors of the melanoma patients. This suggests that there is incomplete central tolerance to these antigens. MART-1, gp100, tyrosinase, and tyrosinase-related protein (TRP) 12 are the common MDAs. Vaccines derived from these proteins have been tested in clinical studies.
Overexpressed antigens are also potential sources of tumor immunotherapeutic vaccines. In comparison to normal cells, some genes are upregulated in tumor cells, ultimately resulting in overexpressed proteins. Peptides derived from these overexpressed proteins (e.g., Her2, MUC1, PRAME, survivin, and telomerase) can be recognized by specific T lymphocytes. This suggests that there is the potential for the development of promising cancer vaccines from them that can be used in the clinic. More specifically, ERBB2, Wilms tumor 1 (WT1), and mucin1 (MUC1) are all examples of these overexpressed antigens.
10.3.2 Single and Polyepitope Vaccines
The simple generations of peptide vaccines only involved single HLA-class I-restricted peptides. Polyepitope vaccines have been developed to enhance the clinical efficiency of vaccines. This is because tumors express multiple tumor antigen epitopes recognized by T cells and a single peptide vaccine targeting only one of them is often inefficient. Simple polyepitope vaccines contain several HLA-class I-restricted peptides from the same HLA type, such as HLA-A24-restricted LY6K-177 and VEGFR1-1084 peptides [10]. Personalized peptide vaccination (PPV) approach with a maximum of four HLA-matched vaccine peptides adopted was developed according to the finding that peptide-specific cellular responses could be detected from the peripheral blood of patients with tumors [11, 12]. A series of Phase I and II PPV clinical trials were performed and showed improved antigen-specific CTL and antibody responses and better clinical outcomes in patients with advanced tumors, such as melanoma, gastric, lung, and prostate cancers [13–16].
It is well-known that Th1 cells promote CTL cell survival and memory response, ultimately resulting in effective CTL-mediated antitumor responses [17]. Simultaneously, tumor-specific T-helper cells can induce tumor senescence and possess direct either antitumor and/or antiangiogenic effects [18, 19]. To this end, a series of studies were performed to study whether peptide vaccines could induce CD4+ T-cell responses. For example, 6 HLA-DR-restricted melanoma helper peptides (6MHP) were used in patients with metastatic melanoma. Results showed that median survival was significantly longer for vaccinated patients. Moreover, that appearance of a specific Th1-dominant CD4+ T-cell response was related with enhanced survival in vaccinated patients [20].
Vaccination using long peptides with both CD8+ and CD4+ epitopes is another proposed strategy for inducing an antitumor response in patients with tumors. Some clinical trials using long peptide cancer vaccines for malignancies have been conducted. For instance, GV1001 is a peptide vaccine with a 16-aa human telomerase reverse transcriptase (hTERT) sequence that can be loaded into multiple HLA class II molecules and may bind the putative HLA class I molecules [21, 22]. A GV1001 Phase II trial in non-small cell lung cancer (NSCLC) patients who had been vaccinated after receiving chemoradiotherapy showed a good toleration to the vaccine. Importantly, it immunized the majority (80%) of NSCLC patients and established a durable T-cell memory [23]. GV1001 vaccination in patients with advanced stage IV melanoma revealed a peptide-specific immune response in 78% (18 out of 23) of the evaluated patients [24].
However, some clinical trials have failed to show effective results in patients with certain types of cancers. For example, a study was performed to explore the safety and immunogenicity in non-resectable pancreatic carcinoma patients using GV1001 vaccination with chemotherapy. Although the combined therapy was safe, the immune responses weren’t strong and lasting enough [25]. In a separate study, six cutaneous T-cell lymphoma (CTCL) patients were vaccinated with the GV-peptide. Unfortunately, none demonstrated objective clinical responses to the vaccination [26]. Taken together, it is clear that further studies need to be conducted to definitively state the effectiveness of a GV1001vaccination in cancer. It should also be noted that other studies using both short- and long-peptide vaccinations aimed at inducing both CD4+ and CD8+ responses have been performed [27, 28].
10.3.3 Clinical Application of Vaccination with TAA Peptides in Gastric Cancer
10.3.3.1 Lymphocyte Antigen 6 Complex Locus K (LY6K)
LY6K has been revealed as a tumor-associated antigen in various tumor types, including those found in bladder, breast, and lung cancers. To this end, it has been reported that vaccine therapy with peptide LY6K-177 stimulated an antigen-specific CD8+ CTL response in patients with esophageal carcinoma [29]. This expression was also immunohistochemically identified in about 85% of gastric cancer samples [30]. Given this past work, a Phase I clinical trial featuring vaccination with HLA-A*2402-restricted LY6K-derived peptide LY6K-177 was conducted at Kinki University in patients with advanced or recurrent gastric cancer [30]. Methodologically, LY6K-177 peptide was mixed with incomplete Freund’s adjuvant ISA 51 and administered intracutaneously to either the inguinal region or axilla of patients. There was no evidence of systemic toxicology during the observation period, suggesting that the peptide vaccine was safe. Three out of six patents achieved a stable disease (SD) clinical response. Moreover, the presence of more specific spots in an ELISPOT assay was correlated with increased survival rates [30].
10.3.3.2 MAGE-A3
There is positive expression of melanoma antigen-A3 genes (MAGE-A3) in over 50% of gastric cancer tumor tissue [31, 32]. Given this, vaccination of dendritic cells (DCs) loaded with both HLA-A2-restricted peptide MAGE-A3-271 and HLA-A24-restricted peptide MAGE-A3-195 derived from MAGE-A3 was tested in the patients with gastrointestinal carcinomas. Postvaccination, peptide-specific CTL responses were detectable in 50% (4/8) of patients. Moreover, tumor markers declined in seven patients. These results suggest that the treatment is a safe and promising immunotherapeutic method for gastrointestinal carcinomas [33].
10.3.3.3 HER-2
HER-2is a transmembrane tyrosine-specific kinase. It is overexpressed at a median rate of 18% (range, 4–53%) of gastric cancers [34]. A Phase I vaccination trial in patients with gastric cancer via DCs pulsed with HLA-A2-restricted HER-2-derived peptide HER2-369 showed that no serious adverse effects were observed in the patients who had received the vaccination. Furthermore, HER2-369 peptide-specific CTL was shown in six of nine patients after immunization. Of these, one patient underwent a partial clinical response and another showed stabilized disease status [35].
10.3.3.4 VEGFR1 and VEGFR2
Vascular endothelial growth factors (VEGFs) are highly specific mitogens for vascular endothelial cells and important in tumor angiogenesis and vasculogenesis. VEGF receptors (VEGFRs) bind different VEGFs and result in different biological responses, which can include angiogenesis, lymphangiogenesis, and inflammatory cell recruitment [36]. As such, both VEGFR1 and VEGFR2 are important antiangiogenic targets. A Phase I/II study of HLA-A24-restricted peptide vaccines used VEGFR1-1084 and VEGFR2-169 in combination with therapeutic agents S-1 and cisplatin in advanced or recurrent gastric cancer patients. Results indicated that this combined approach was both safe and highly effective to patients with either advanced or recurrent gastric cancer. More specifically, VEGFR1-1084- and VEGFR2-169-specific CTL responses were each induced in 82% (18/22) of patients. Of those tested, twelve patients demonstrated a partial response, while ten showed a stable disease status after two cycles of the combination treatment. Collectively, these results suggest that this combined strategy gives promise for the therapy of advanced cancers.
10.3.3.5 LY6K and VEGFR1
A Phase I trial of a peptide vaccination with HLA-A24-restricted LY6K-177 and VEGFR1-1084 peptides in patients with chemotherapy-resistant, advanced, and unresectable gastric cancer showed that such vaccination was safe for advanced gastric cancer. Four out of 12 patients had a stable disease state after a single treatment course in this study [10].
10.3.3.6 WT1 and MUC1
HLA-A2402-restricted Wilms’ tumor gene WT1-modified peptide (CYTWNQMNL) has an amino-acid mutation (M to Y at position 2) to increase the binding affinity to HLA-A*2402 molecules. With this substitution, WT1-specific CTL was more effectively elicited than if the wild-type peptide from PBMC of HLA-A*2402-positive healthy volunteers was used [37]. To this end, vaccination of DC pulsed with MUC1 long peptide (TRPAPGSTAPPAHGVTSAPDTRPAP-GSTAP) was safely used in patients with recurrent ovarian cancer [38]. Furthermore, vaccination of DC pulsed with WT1 peptide and MUC1 long peptide was used in a patient with locally recurrent gastric cancer. The patient had been in remission for 30 months, suggesting the therapeutic benefit of intratumoral injections of DCs into the patients who could not undergo endoscopic submucosal dissection or surgery [39].
10.3.3.7 Personalized TAA Peptide Vaccination
Vaccinations can be made by screening personalized peptides according to preexisting T-cell responses to the given peptide. Patients with advanced gastric cancer were screened using a 16-peptide library for HLA-A2 allele and a 14-peptide library for HLA-A24 allele. Enhanced T-cell and antibody responses to the screened peptides were detected. Crucially, patients with the immune responses to the vaccinated peptides showed prolonged survival [14]. Another study featuring personalized peptide vaccinations with TS-1was done for advanced gastric and/or colorectal carcinoma patients. This study also showed that the combination treatment was well tolerated. Moreover, postvaccination peptide-specific IgG and interferon-gamma production by CTL were both increased in 9/11 and 7/11, respectively [40].
10.4 Neoantigen-Based Tumor Vaccines
10.4.1 Introduction
Over the course of its evolution, the immune system has developed to recognize and destroy foreign invaders. Given this basis, the foundation of a successful immunotherapy is inherently an issue of “self” versus “non-self” discrimination [41]. Until recently, the majority of researches in tumor immunotherapy were placed on tumor-associated antigens and shared antigens expressed in both tumors and normal tissues [42, 43]. However, the low affinity to TCRs resulting from incomplete central tolerance and on-target but off-tumor toxicity limits the application of these antigens. To this end, work in transgenic mice with non-mutated, tumor antigen-specific TCR expressed in all T cells showed they were unable to reject tumors that express the P1A antigen [43]. Highly specific tumor antigens that are displayed exclusively by tumor cells without normal cell expression have the potential to elicit a robust tumor-specific, immune response. This specificity is theorized to result in minimal risk for adverse effects, thus making it a promising target for cancer immunotherapies such as therapeutic vaccines and engineered T cells [44, 45]. On theoretical grounds, cancer rejection epitopes may be derived from one of two antigen classes. A first class of potential cancer rejection antigens is formed by non-mutated proteins to which T-cell tolerance is incomplete—for instance, because of their restricted tissue expression pattern, as seen in cancer-testis antigens (CTAs). A second class of potential cancer rejection antigens is derived from gene segments which are completely absent in normal human genome and include both viral and neoantigens [44]. Among these classes of cancer-specific antigens, neoantigens have received the most attention since they are taken to be strictly tumor specific. With this in mind, scientists have sought to harness this specificity to draw the cancer out of its cloak of immune tolerance. In this manner, it would be visible, allow for a provocation of a T-cell response, and putatively lead to cancer control or eradication.
When compared with non-mutated self-antigens, it has been proposed that neoantigens are more intimately correlated with tumor control [46]. Possible reasons for this are as follows: (1) some somatic mutations may serve as core steps in the process of oncogenesis and could influence or decide biological tumor behaviors [47]; (2) neoantigens are expressed exclusively in tumor tissue and therefore minimize the risk of on-target, off-tumor killing of healthy tissue; (3) finally, given that neoantigens are derived from mutations acquired during in tumorigenesis, the TCR repertoire of T-cell progenitors has not encountered neoantigens during thymic development. In theory, this would lead to a lack of central tolerance toward neoantigens. Neoantigen-specific T cells should not be deleted by negative selection and may be present in circulation [48].
It is known that gastric cancers display a high somatic mutational burden, with only melanoma, lung, and bladder cancers displaying a more mutated profile [47]. There are genetic and etiological features specific to gastric cancers, which may have relevance when considering their suitability for immune-targeting approaches.
In the past two decades, a cDNA library was frequently utilized in screening for unique neoantigens [49]. The classical cDNA library screening approach, which led to the discovery of multiple neoantigens, is a labor-intensive method that inherently low throughput. Collectively, this can lead to failures in identifying some mutated antigens. A large fraction of the mutations found in human tumors is patient specific, meaning that they are not shared between patients. Therefore, technologies that are designed to analyze T-cell reactivity against putative mutation-derived neoantigens should be based on the genome of an individual tumor [44]. Recently, the development of whole-exome sequencing technologies has made the identification of non-synonymous tumor mutations easier and more efficient. To this end, mutated peptide immunogenicity was analyzed using a prediction algorithm. The potential neoantigens that resulted from this analysis underwent further experiments to investigate their inherent T-cell reactivity [50, 51].
The application of modern sequencing technology to the development of personalized neoantigen-based vaccines represents an exciting fusion of genomics and immunotherapy, with potentially important clinical implications for gastric cancer treatment.
10.4.2 Evidence That Neoantigens Are Dominant Tumor Regression Antigens
Long-term follow-up studies have shown that a substantial subset of patients receiving tumor-infiltrating lymphocytes (TILs) for metastatic melanoma experienced complete, lasting, and even curative tumor regression [52]. This field was pioneered by Steven Rosenberg of National Cancer Institute (NCI), whose team developed methods for the isolation and culture of tumor-infiltrating lymphocytes (TILs). After lymhodepleting chemotherapy, TILs were reinfused together with exogenous IL2 to heavily pretreat patients with metastatic melanoma. This approach resulted in objective clinical responses in 52/93 patients (56%) and complete responses in 20/93 patients (22%) [53]. Other medical centers, such as the MD Anderson Cancer Center in Houston, TX, USA, had overall responses in 13/31 patients (42%) [54].
Recent studies have more specifically investigated the tumor antigens recognized by TILs and the probable specificities of the T cells mediating tumor rejection. Early studies identified TILs and other T cells in melanoma with reactivity against the shared tumor/self-melanocyte differentiation antigens (MDAs) (e.g., gp100, MART1, tyrosinase) [52]. However, both melanomas and normal melanocytes in skin and retina express MDAs. Given this, autoimmune toxicities against these normal tissues were rarely observed—even in patients who experienced profound tumor regression. The frequency of MDA-specific T cells in bulk TIL populations was also generally low [55, 56]. Conversely, TCR T-cell therapies that specifically target MDAs with high affinity have been shown to consistently induce autoimmune toxicities and rarely mediate tumor regression [57]. Therefore, the primary approach for TIL antitumor effects should not be ascribed to MDA-specific T cells. In reality, increasing evidence has suggested that neoantigens derived from genetic mutations are the main targets of tumor-reactive TILs and the primary cancer rejection antigens in melanoma. These mutations result in neoepitopes which can be recognized by TILs. Moreover, it is possible that T cells with specificity for these neoepitopes constitute the dominant tumor-reactive populations in TILs [58, 59].
Unlike self-antigens such as MDAs, T-cell affinity for mutated epitopes is not limited by thymic negative selection. TIL clones against mutated gene products routinely display high target antigen avidity and robust recognition of autologous tumor lines [58, 59]. It seems likely, therefore, that melanoma immunogenicity is linked to the high frequency of mutational events in this cancer. Moreover, that T cells specific for mutated gene products are responsible for tumor regression in patients receiving TIL therapy.
Checkpoint inhibitor therapy, such as anti-CTLA-4 and/or anti-PD-1 monoclonal antibody, blocks the inhibitory signals toward T cells. It is a revolutionary cancer treatment and has achieved significant clinical benefits including robust and long-lasting responses in several different malignancies [60–63]. Mechanistically, such checkpoint agents activate the immune system in order to attack tumor cells. To this end, Gubin and colleagues used genomics and bioinformatics approaches to identify neoantigens derived from tumor-specific mutant proteins as a major class of T-cell rejection antigens. This was done following anti-PD-1 and/or anti-CTLA-4 therapy in a sarcoma mouse model. Furthermore, they showed that therapeutic synthetic peptide vaccines incorporating these mutant epitopes induced tumor rejection to levels comparable to those of checkpoint blockade immunotherapy.
It has been recently proposed that the mutation load and neoantigen landscape might serve as biomarkers to predict clinical responsiveness to checkpoint blockade therapy, given that a mutational gene signature was inferred to correlate with the long-term clinical benefits seen in anti-CTLA-4 therapy [64]. Recently, Rizvi and colleagues used whole-exome sequencing of non-small cell lung cancers treated with anti-PD-1 (pembrolizumab). Their results confirmed that they had a higher, non-synonymous mutation burden in tumors associated with improved objective response, robust clinical benefit, and progression-free survival [65]. Subsequently, a separate study enrolling more than 100 patients with metastatic melanoma confirmed that those with the highest neoantigen load were most likely to respond to ipilimumab [66, 67]. Following this study, Diaz, Jr. et al. [68] found that colorectal cancers that had a large number of somatic mutations caused by mismatch-repair defects were susceptible to immune checkpoint blockade. For mismatch repair–deficient colorectal cancers, the immune-related objective response and immune-related progression-free survival rates were 40% and 78%, respectively. For mismatch repair–proficient colorectal cancers, the rates were 0% and 11%, respectively. Whole-exome sequencing revealed a mean of 1782 somatic mutations per tumor in mismatch repair–deficient colorectal cancers, compared with 73 in mismatch repair–proficient tumors. High somatic mutational loads were associated with prolonged progression-free survival.
Taken together, these studies revealed that neoantigens are not only important targets for checkpoint blockade therapy but that they can also be used to develop personalized, cancer-specific vaccines. Mechanistically, they can also be used to probe the underpinnings of different checkpoint blockade treatments. Indeed, neoantigen-specific T cells have been shown to underlie the clinical responses to many current standard treatments and immunotherapeutic interventions.
10.4.3 Neoantigen Identification
Tumor-specific mutations are ideal targets for cancer immunotherapy, as they lack expression in healthy tissues and can potentially be recognized as neoantigens by the mature T-cell repertoire. The predominant obstacle for the clinical use of neoantigen-based vaccine approaches is the fact that every patient’s tumor possesses a unique set of mutations (“the mutanome”). In order for such a therapeutic approach to be effective, each mutanome must first be identified. Over the past two decades, classical cDNA library screening has been utilized to screen neoantigens. In this approach, cDNA library and MHC molecules were overexpressed in cell lines and then cocultured with T cells. This was done to identify antigens that could successfully induce T-cell activation, which was monitored by up-regulation of cytokine secretion and active markers [49]. The development of exome sequencing and bioinformatics has allowed for significant technical advances in the rapid identification for personalized cancer neoantigens. As such, they have intrigued immunologists into targeting these mutations for cancer immune therapy.
To this end, the Rosenberg research group at the National Cancer Institute has developed a screening approach involving mining whole-exome sequence data and MHCI/peptide bind prediction algorithm to identify neoantigens expressed in patients with melanoma [59]. Using this approach, they have successfully identified mutated antigens expressed on autologous tumor cells that were recognized by three bulk TIL lines from three individuals with melanoma that were associated with objective tumor regressions following adoptive cell transfer. However, this approach is limited by the accuracy of the predictive algorithms that are used. At times, these algorithms can be adequate, sometimes even resulting in excessively large candidate numbers. Yadav and colleagues developed a screening approach that combines whole-exome and transcriptome sequencing analysis with mass spectrometry to identify neoepitopes in two widely used murine tumor models [69]. Their predictions lead to the generation of peptide-MHC I dextramers, enabling the monitoring of the kinetics and distribution of antitumor T-cell responses both before and after vaccination. Moreover, Kalaora and colleagues [70] also reported on a combined whole-exome sequencing and mass spectrometry method to analyze the mutated HLA-I peptidome of human melanoma cells.
Another method is the use of tandem minigenes (TMGs) that encode polypeptides containing a mutated amino acid residue flanked on their N- and C-termini by 12–13 amino acids. The previous work synthesized tandem minigene constructs, which were then used to transfect autologous APCs or cell lines co-expressing autologous HLA molecules. Using this method, Rosenberg and colleagues reported a patient with widely metastatic cholangiocarcinoma whose lung metastases were resected and adopted as a source for WES. This revealed 26 non-synonymous mutations [71]. Finally, they constructed three TMGs that covered all 26 non-synonymous mutations and demonstrated that TILs from this metastatic cholangiocarcinoma patient contained CD4+ T cells that recognized a mutation in erbb2 interacting protein (ERBB2IP) expressed by the cancer. Using NGS combined with a high-throughput, immunological screening approach constructed of tandem minigenes, they demonstrated that TILs from 9/10 patients with metastatic gastrointestinal cancers contained CD4+ and/or CD8+ T cells that recognized one to three neoepitopes derived from patient-specific somatic mutations [72].
10.4.4 MHC II-Restricted Neoantigens and CD4+ T Cells in Antitumor Immunity
Given that CD8+ CTLs can directly kill tumor cells and destroy tumor masses in vivo, much attention has been paid to the role of CD8+ T cells in tumor immunotherapy. To this end, a series of MHC-class I-restricted TAs have been selected and identified. Although antigen-specific CD8+ T cells are widely considered to be superior, there are many advantages to CD4+ T-cell-mediated responses to tumor antigens over CD8+ T cells [73]. These advantages are as follows: (1) as opposed to the restricted HLA type of CD8+ T-cell-mediated immunity, CD4+ T-cell-mediated immunity is much more HLA promiscuous; (2) independent activation of CD4+ T cells relative to antigen presentation by tumor cells, meaning there is no dampening due to their impaired presentation machinery; (3) CD4+ T cells can play a supporting role like T helper cells; (4) CD4+ T cells are able to directly mediate cytotoxicity, in an IFN-γ and MHC-class II-restricted way that is independent of either CD8+ T or NK cells in host; (5) tumor cells can process and present MHC class II epitopes by autophagy; (6) IFN-γ can induce up-regulation of MHC class II in tumor cells, thus rendering them susceptible to CD4+ T-cell-mediated cytotoxicity [18]; (7) CD4+ Th cells secrete multiple cytokines once antigens are encountered, thereby inducing an inflammatory microenvironment that can provide a co-stimulatory signal for innate and adaptive immune cells; finally, (8) CD4+ T cells help CD8+ T cells to proliferate, maintain their function, and infiltrate tumors.
As mentioned above, MHC class II-restricted neoantigens and neoantigen-reactive CD4+ T cells have the potential to not only realize a broader range of immune responses but also a stronger and longer-lasting immune response when compared with single MHC class I-restricted neoantigens. Tools for identifying MHC class I molecule restricted neoantigens are relatively efficient. However, the algorithms to predict epitopes that bind to MHC class II molecules and engage receptors on CD4+ T cells are far less accurate [67]. More recently, Sahin et al. conducted an experiment that found 80–90% of immunogenic neoepitopes were recognized by CD4+ T rather than CD8+ T cells. This demonstrated that personalized tumor mutant neoantigens are perhaps more likely to bind to MHC II molecules [74]. They then established a process by which mutations screened out by exome sequencing could be identified as vaccine targets simply through bioinformatic prioritization combining expression level and major histocompatibility complex (MHC) class II-binding capacity of neoantigens. Thus, this would enable the production of poly-neoepitope messenger RNA vaccines much more conveniently and rapidly.
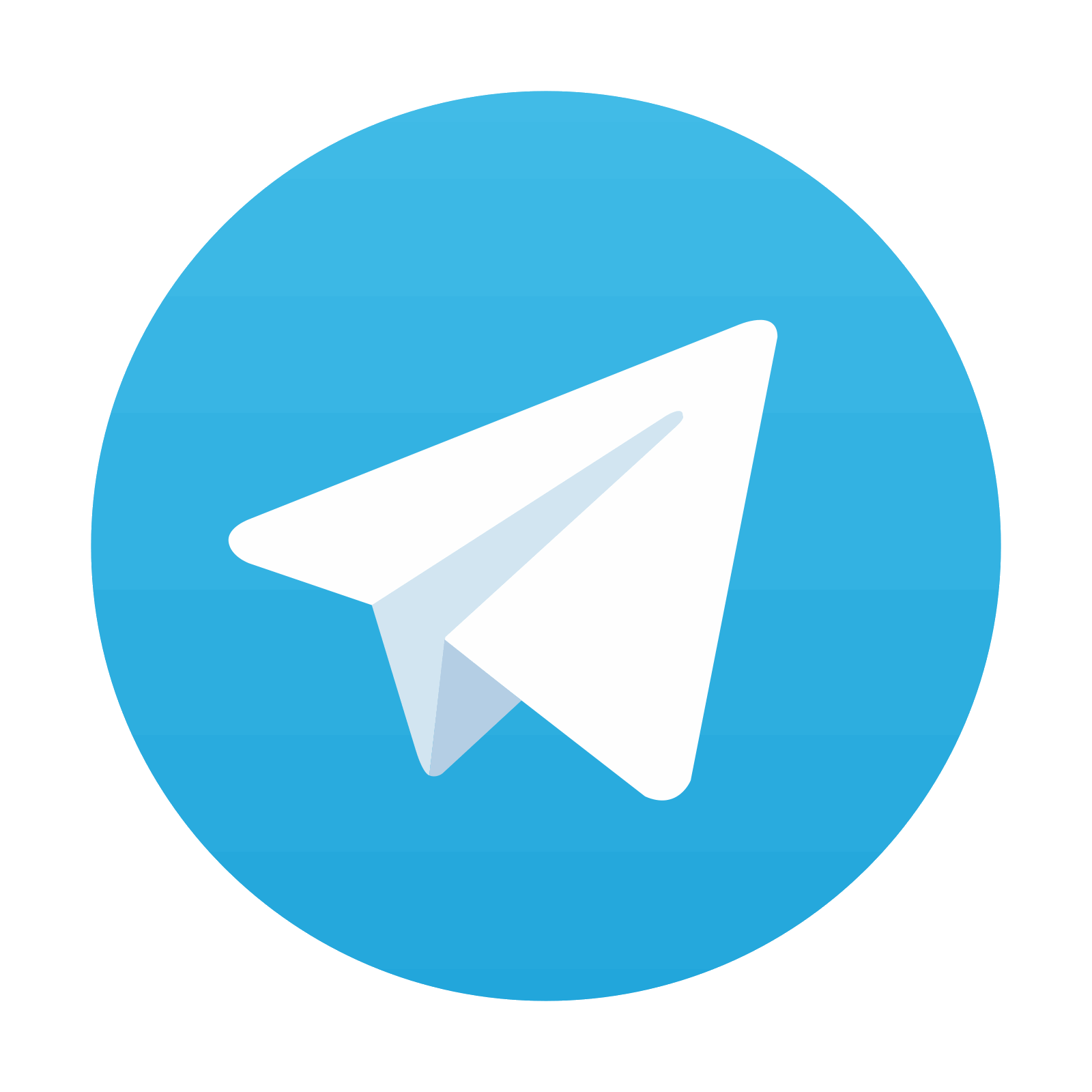
Stay updated, free articles. Join our Telegram channel
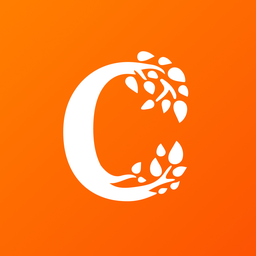
Full access? Get Clinical Tree
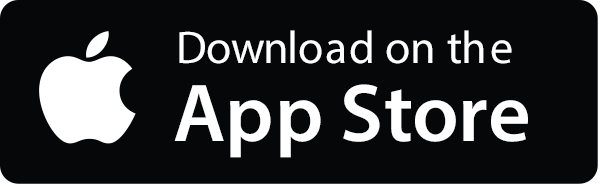
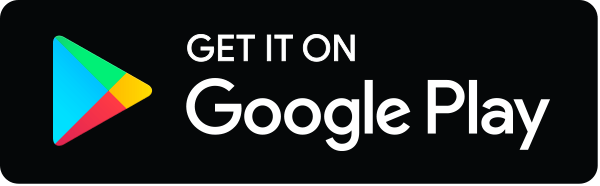