Clonal myeloid disorders are characterized by genetic alterations that activate cytokine signaling pathways and stimulate cell proliferation. These activated signaling pathways have been extensively studied as potential therapeutic targets, and tyrosine kinase inhibitors have indeed had extraordinary success in treating BCR/ABL-positive chronic myeloiud leukemia. However, although inhibitors of other activated kinases have been developed that perform well in preclinical studies, the therapeutic efficacy of these drugs in patients has been unimpressive. This article discusses potential reasons for these discordant results and outlines recent scientific advances that are informing future efforts to target activated kinases in clonal myeloid disorders.
Key points
- •
Clonal myeloid diseases include the myeloproliferative neoplasms: chronic myeloid leukemia (CML), polycythemia vera (PV), essential thrombocythemia (ET), primary myelofibrosis (PMF), chronic eosinophilic leukemia (CEL), and systemic mastocytosis (SM); and acute myeloid leukemia (AML).
- •
Clonal myeloid diseases have in common the mutational activation of cellular kinases that play an important role in driving aberrant cell proliferation.
- •
Therapeutic targeting of activated cellular kinases has had mixed results in the treatment of clonal myeloid disorders.
- •
The variability in efficacy of kinase-targeted therapies is due to several different factors, including the feasibility of kinase targeting, the on-target and off-target toxicity of kinase targeting, and the extent to which different clonal myeloid disorders are “addicted” to their activated kinases.
Introduction
Clonal myeloid diseases are a group of acquired hematopoietic stem cell disorders that are characterized by clonal proliferation of 1 or more myeloid cell lineages. Acute myeloid leukemia (AML) results from the uncontrolled proliferation of abnormal myeloid progenitor cells, whereas myeloproliferative neoplasms (MPN) are characterized by excessive production of mature blood cells: neutrophils (in chronic myeloid leukemia [CML]), erythrocytes (in polycythemia vera [PV]), megakaryocytes (in essential thrombocythemia [ET] and primary myelofibrosis [PMF]), eosinophils (in chronic eosinophilic leukemia [CEL]) and mast cells (in systemic mastocytosis [SM]). Except in the case of PMF, cellular maturation in MPN proceeds normally and the expanded cell populations are functionally intact. In PMF, megakaryocyte differentiation is dysregulated and is thought to trigger the release of cytokines that, induce reactive bone marrow fibrosis and extramedullary hematopoiesis. MPN and AML are driven by diverse genetic alterations but they have in common the mutational activation of cellular kinases, and dysregulated cytokine signaling has been found to play a key role in the pathophysiology of clonal myeloid disorders. This has provided a strong rationale for the therapeutic targeting of dysregulated kinase signaling to treat MPN and AML.
CML is emblematic of the promise of kinase inhibitors as anticancer therapy. CML is an indolent leukemia with a high rate of progression to “blast-phase CML,” a highly aggressive acute leukemia with a particularly poor prognosis. The genetic driver in CML is a fusion oncoprotein, BCR-ABL, that is the product of a balanced translocation between chromosomes 9 and 22 that gives rise to the “Philadelphia chromosome.” BCR-ABL has constitutive Abelson (ABL) tyrosine kinase activity, and the proliferation and survival of CML cells is absolutely dependent on this kinase activity. This makes BCR-ABL’s role in CML a paradigm of “oncogene addiction.” Because of their oncogene-addicted state, CML cells are exquisitely sensitive to tyrosine kinase inhibition, and tyrosine kinase inhibitors (TKI) have revolutionized the treatment of CML. A previously near-universally fatal malignancy has been transformed into a chronic disease with long-term remission rates of more than 90%.
TKI therapy is the culmination of 4 decades of intensive laboratory investigation. The Philadelphia chromosome was discovered by Peter Nowell and David Hungerford in 1960. This was followed by discovery of the t(9;22) chromosomal translocation by Janet Rowley in 1973, characterization of the BCR-ABL fusion protein by Nora Heisterkamp and John Groffen in 1985, confirmation of the in vitro oncogenic activity of BCR-ABL by Owen Witte in 1987, and validation of the ability of BCR-ABL to induce CML in vivo by George Q. Daley and Richard Van Etten in 1999. In 1996, Brian Druker and colleagues identified a specific inhibitor of Abl kinase activity, CGP-57148, that selectively kills BCR-ABL–transformed cells. In 2001, CGP-57148, better known as imatinib, received accelerated Food and Drug Administration (FDA) approval for the treatment of CML.
The discovery of the Philadelphia chromosome in 1960 to the FDA approval of imatinib in 2001 took 40 years. In the modern era, this timeline has been dramatically shortened and the time from discovery of a genetic lesion to first-in-human clinical trials is steadily decreasing. For example, in 2005, the activating JAK2 mutation JAK2V617F was found to be highly recurrent in PV, ET, and PMF. Just 6 years later, in 2011, the first-in-class JAK2 inhibitor, Ruxolitinib, was approved for use in patients. Fig. 1 outlines the time from identification of specific clonal myeloid disease mutations to clinical targeting of the mutations in patients. This accelerated timeline reflects, in part, the fact that a number of previously developed drugs are being repurposed to target other oncogenic kinases. For example, Dasatinib, a TKI that was originally engineered to target BCR-ABL, was found to have in vitro activity against the stem cell factor receptor tyrosine kinase (KIT) mutation KITD816V, an imatinib-resistant activating mutation in KIT that is present in more than 80% of cases of SM. Several other factors have contributed to this recent acceleration in target discovery and drug development, including large-scale sequencing efforts in cancer, improved technologies that allow for more rapid building of in vitro and in vivo models, and industrialized high-throughput compound screening.
Inspired by the success of TKIs in CML, laboratories around the world have for years been working to target other oncogenic enzymes in other cancers. Although there have been a number of notable success stories, including epidermal growth factor receptor (EGFR) inhibitors for the treatment of EGFR-mutant non–small-cell lung cancer (NSCLC), BRAF and mitogen-activated protein kinase-kinase (MEK) inhibitors for the treatment of BRAF-mutant melanoma, and anaplastic lymphoma kinase (ALK) inhibitors for the treatment of ALK-rearranged NSCLC, none of these drugs has had the profound therapeutic efficacy of TKIs in CML. Even in other hematologic malignancies, targeted therapies have, for the most part, fallen short. JAK inhibitors in JAK2-mutant PV, ET, and PMF, and FLT3 inhibitors in FLT3-mutant AML, do have antidisease activity, but they have not been able to induce sustained remissions or to significantly prolong survival of patients with these diseases.
This introductory article explores reasons why, despite major advances in our understanding of the pathophysiology of clonal myeloid diseases, and despite the accelerated translation of biological findings into therapeutic agents, efforts to target oncogenic kinases in myeloid malignancies have been largely disappointing. We ask what lessons can be learned from these setbacks and discuss alternative therapeutic approaches that are currently being explored that may lead to more successful targeting of signaling pathways in clonal myeloid disorders and in cancer in general.
Introduction
Clonal myeloid diseases are a group of acquired hematopoietic stem cell disorders that are characterized by clonal proliferation of 1 or more myeloid cell lineages. Acute myeloid leukemia (AML) results from the uncontrolled proliferation of abnormal myeloid progenitor cells, whereas myeloproliferative neoplasms (MPN) are characterized by excessive production of mature blood cells: neutrophils (in chronic myeloid leukemia [CML]), erythrocytes (in polycythemia vera [PV]), megakaryocytes (in essential thrombocythemia [ET] and primary myelofibrosis [PMF]), eosinophils (in chronic eosinophilic leukemia [CEL]) and mast cells (in systemic mastocytosis [SM]). Except in the case of PMF, cellular maturation in MPN proceeds normally and the expanded cell populations are functionally intact. In PMF, megakaryocyte differentiation is dysregulated and is thought to trigger the release of cytokines that, induce reactive bone marrow fibrosis and extramedullary hematopoiesis. MPN and AML are driven by diverse genetic alterations but they have in common the mutational activation of cellular kinases, and dysregulated cytokine signaling has been found to play a key role in the pathophysiology of clonal myeloid disorders. This has provided a strong rationale for the therapeutic targeting of dysregulated kinase signaling to treat MPN and AML.
CML is emblematic of the promise of kinase inhibitors as anticancer therapy. CML is an indolent leukemia with a high rate of progression to “blast-phase CML,” a highly aggressive acute leukemia with a particularly poor prognosis. The genetic driver in CML is a fusion oncoprotein, BCR-ABL, that is the product of a balanced translocation between chromosomes 9 and 22 that gives rise to the “Philadelphia chromosome.” BCR-ABL has constitutive Abelson (ABL) tyrosine kinase activity, and the proliferation and survival of CML cells is absolutely dependent on this kinase activity. This makes BCR-ABL’s role in CML a paradigm of “oncogene addiction.” Because of their oncogene-addicted state, CML cells are exquisitely sensitive to tyrosine kinase inhibition, and tyrosine kinase inhibitors (TKI) have revolutionized the treatment of CML. A previously near-universally fatal malignancy has been transformed into a chronic disease with long-term remission rates of more than 90%.
TKI therapy is the culmination of 4 decades of intensive laboratory investigation. The Philadelphia chromosome was discovered by Peter Nowell and David Hungerford in 1960. This was followed by discovery of the t(9;22) chromosomal translocation by Janet Rowley in 1973, characterization of the BCR-ABL fusion protein by Nora Heisterkamp and John Groffen in 1985, confirmation of the in vitro oncogenic activity of BCR-ABL by Owen Witte in 1987, and validation of the ability of BCR-ABL to induce CML in vivo by George Q. Daley and Richard Van Etten in 1999. In 1996, Brian Druker and colleagues identified a specific inhibitor of Abl kinase activity, CGP-57148, that selectively kills BCR-ABL–transformed cells. In 2001, CGP-57148, better known as imatinib, received accelerated Food and Drug Administration (FDA) approval for the treatment of CML.
The discovery of the Philadelphia chromosome in 1960 to the FDA approval of imatinib in 2001 took 40 years. In the modern era, this timeline has been dramatically shortened and the time from discovery of a genetic lesion to first-in-human clinical trials is steadily decreasing. For example, in 2005, the activating JAK2 mutation JAK2V617F was found to be highly recurrent in PV, ET, and PMF. Just 6 years later, in 2011, the first-in-class JAK2 inhibitor, Ruxolitinib, was approved for use in patients. Fig. 1 outlines the time from identification of specific clonal myeloid disease mutations to clinical targeting of the mutations in patients. This accelerated timeline reflects, in part, the fact that a number of previously developed drugs are being repurposed to target other oncogenic kinases. For example, Dasatinib, a TKI that was originally engineered to target BCR-ABL, was found to have in vitro activity against the stem cell factor receptor tyrosine kinase (KIT) mutation KITD816V, an imatinib-resistant activating mutation in KIT that is present in more than 80% of cases of SM. Several other factors have contributed to this recent acceleration in target discovery and drug development, including large-scale sequencing efforts in cancer, improved technologies that allow for more rapid building of in vitro and in vivo models, and industrialized high-throughput compound screening.
Inspired by the success of TKIs in CML, laboratories around the world have for years been working to target other oncogenic enzymes in other cancers. Although there have been a number of notable success stories, including epidermal growth factor receptor (EGFR) inhibitors for the treatment of EGFR-mutant non–small-cell lung cancer (NSCLC), BRAF and mitogen-activated protein kinase-kinase (MEK) inhibitors for the treatment of BRAF-mutant melanoma, and anaplastic lymphoma kinase (ALK) inhibitors for the treatment of ALK-rearranged NSCLC, none of these drugs has had the profound therapeutic efficacy of TKIs in CML. Even in other hematologic malignancies, targeted therapies have, for the most part, fallen short. JAK inhibitors in JAK2-mutant PV, ET, and PMF, and FLT3 inhibitors in FLT3-mutant AML, do have antidisease activity, but they have not been able to induce sustained remissions or to significantly prolong survival of patients with these diseases.
This introductory article explores reasons why, despite major advances in our understanding of the pathophysiology of clonal myeloid diseases, and despite the accelerated translation of biological findings into therapeutic agents, efforts to target oncogenic kinases in myeloid malignancies have been largely disappointing. We ask what lessons can be learned from these setbacks and discuss alternative therapeutic approaches that are currently being explored that may lead to more successful targeting of signaling pathways in clonal myeloid disorders and in cancer in general.
What makes a good drug-target pairing?
TKIs have been successful in the treatment of CML for clearly identifiable reasons. First and foremost, the ABL kinase domain is highly amenable to drug targeting. Second, the pathways that are activated by BCR-ABL play an essential role in the pathophysiology of CML. Third, inhibition of ABL does not result in unacceptable toxicity. Several decades of work identifying, validating, and targeting oncogenic enzymes has taught us that, for a drug-target pairing to be truly successful, all 3 of these criteria must be met. Although this may seem, at first glance, to be a straightforward proposition, the reality is that these criteria set a very high bar for success that few drug-target pairings have, to date, been able to achieve.
Drugging the undruggable
Not all cancer-associated activating mutations in signaling proteins are amenable to molecular targeting. A classic example is the RAS family of small GTPases. RAS GTPases are among the most frequently mutated genes in cancer, and more than 10% of AML cases harbor activating RAS mutations. Despite years of research, efforts to directly inhibit RAS have, to date, been unsuccessful. One challenge to targeting RAS is structural. Unlike the ATP-binding domains of tyrosine kinases, which are structured pockets on the surface of the kinases that are amenable to small molecule targeting, the GTP-binding domains of RAS are not clearly defined structures to which small molecules can readily bind with high specificity and high affinity. Another challenge relates to the different mechanisms by which ATP and GTP modulate enzymatic activity. Tyrosine kinases bind ATP with low micromolar affinity and catalyze the rapid transfer of γ-phosphoryl groups from ATP to tyrosine residues on their substrates. This makes it possible for ATP-competitive inhibitors, such as imatinib, to effectively inhibit kinase activity in cells. RAS, on the other hand, binds GTP with picomolar affinity and cycles between a GTP-bound active state and a GDP-bound inactive state, making competitive inhibition of GTP binding to RAS unfeasible. Because of these biophysical constraints, efforts to target RAS have primarily focused on inhibiting functionally important posttranslational modifications of RAS and downstream effectors of RAS, rather than on directly inhibiting RAS itself. For example, the enzyme farnysyltransferase attaches a farnysyl moiety to RAS that facilitates its localization to the inner surface of the plasma membrane, and the membrane localization of RAS has been found to be required for its oncogenic activity. Unfortunately, attempts to target farnysyltransferase in RAS-mutant clonal myeloid diseases have, so far, been disappointing, likely due to the existence of alternative RAS-activating posttranslational modifications, including geranylgeranylation. Another strategy is to target RAS effector pathways. Inhibitors of phosphatidylinositol 3-kinase, MEK, and protein kinase B (AKT) have all been found to have modest antileukemic activity in patients, and efforts to combine these therapies are currently ongoing. However, it is not known whether these drug combinations will be able to inhibit oncogenic RAS signaling sufficiently to induce durable remissions in patients and also have tolerable side-effect profiles.
Oncogene addiction versus disease acceleration
In 2014, 3 groups reported that a significant proportion of older individuals with normal blood counts harbor somatic mutations in their hematopoietic stem cell compartment in genes associated with hematologic malignancies. This subclinical clonal hematopoiesis, termed clonal hematopoiesis of indeterminate potential (CHIP), is associated with an increased risk of developing hematologic malignancies. Prior studies on the mutational hierarchy of AML found that mutations in several epigenetic genes, including TET2 , DNMT3A , and ASXL1 , are likely to be early events in the clonal evolution of AML. Interestingly, these same genes are the most frequently mutated in CHIP. Altogether, these observations suggest that CHIP-associated mutations are “founder mutations” that initiate clonal hematopoiesis, and that CHIP is a premalignant condition.
Interestingly, of the genes associated with CHIP, only JAK2 and CBL function through dysregulation of cytokine signaling. Mutations in other AML-associated kinases, including FLT3, KIT, and platelet-derived growth factor receptor, have not been found in CHIP, which is consistent with studies that suggest that kinase mutations are typically late events in clonal hematopoiesis. This has profound therapeutic implications because even the most potent inhibitor of an oncogenic kinase can only inhibit the proliferation and survival of AML cells that are dependent on that kinase. If only a subset of a patient’s malignant cells is dependent on a kinase, then inhibition of that kinase will leave any nonaddicted cells untouched, and the disease will persist. That is not to say that “late” mutational events are necessarily poor drug targets. If a mutant kinase were to drive a preleukemic clone to become fully transformed, then targeting that kinase could induce a durable clinical remission because the ancestral clone would need to acquire new mutations to mediate disease relapse. On the other hand, targeting a mutant kinase that simply accelerates the proliferation of already fully transformed cells is less likely to be effective. Inhibiting a kinase under these circumstances might eradicate the mutation-positive malignant clone and induce a transient clinical response, but it is unlikely to significantly alter the natural history of the disease.
Efforts to target FLT3 signaling in AML are illustrative of just how complex the interplay between a therapeutic target and its position in the clonal hierarchy of transformation can be. There are 2 major classes of FLT3 mutations in AML, in-frame internal-tandem duplications in the juxtamembrane domain of FLT3, and point mutations in the tyrosine kinase domain of FLT3, both of which result in constitutive activation of FLT3 and activation of downstream signaling pathways. Activating FLT3 mutations are typically late events in the clonal evolution of AML and are associated with increased white blood cell counts, high percentages of peripheral blood and bone marrow blasts, and poor overall survival. Several FLT3 inhibitors have been tested in patients, and response rates as high as 70% have been reported. However, even patients treated with single-agent FLT3 inhibitors who experience a significant reduction in their blast count typically relapse within weeks, and FLT3 inhibitors have not significantly improved outcomes in patients with AML.
Despite the admittedly disappointing results of past clinical trials of FLT3 inhibitors, the specific characteristics of response and relapse in patients have been quite informative. Multiple clinical trials have found a correlation between how potently FLT3 signaling is suppressed in a given patient and their likelihood of having a clinical response. This suggests that, in many patients, inhibiting FLT3 signaling does have antileukemic effects and FLT3 signaling does drive disease. Moreover, many patients with FLT3-mutant AML who initially respond to FLT3 inhibitors relapse with FLT3-mutant disease in which constitutive FLT3 signaling has been restored. This suggests that, in these patients, FLT3 inhibitors are acting “on-target” to drive selection for FLT3 inhibitor-resistance. It also suggests that, despite being a “late” event, activation of FLT3 plays a central role in the pathophysiology of FLT3-mutant AML. However, there are also patients with FLT3-mutant AML who initially respond to FLT3 inhibitor therapy but who then rapidly relapse with FLT3 wild-type disease. This suggests that, in these patients, FLT3 inhibitors are able to successfully suppress or even eradicate FLT3-mutant cells, and FLT3 inhibition selects for coexisting AML cells that do not harbor a FLT3 mutation. So where does this leave mutant FLT3 as a therapeutic target? Is there a way to anticipate the extent to which a given patient’s AML is dependent on FLT3 signaling? Can we predict when FLT3 is truly driving disease and when it is merely favoring the clonal expansion of a specific AML subclone?
There is one additional finding from the FLT3 inhibitor clinical trials that adds yet another layer of complexity to the question of who should be treated with these agents. Several trials have found that therapeutic responses to FLT3 inhibitors are not restricted to patients with FLT3-mutant AML. In fact, many patients with FLT3 wild-type AML have experienced significant, albeit transient, responses to FLT3 inhibitor therapy. These responses could be the result of “off-target” activities of FLT3 inhibitors against other oncogenic pathways. Alternatively, they could signify that wild-type FLT3 signaling plays a role in driving the proliferation and survival of AML cells irrespective of their FLT3 mutational status. If so, should all patients with AML be treated with FLT3 inhibitors? Should the goal of FLT3 inhibitor therapy be complete suppression of both wild-type and mutant FLT3 signaling? How would such a therapy be tolerated from a toxicity standpoint? One clue to this last question may be gleaned from mouse models. Mice with complete genetic deletion of FLT3 have reduced numbers of B-cell precursors and defective dendritic cell function, and their hematopoietic stem cells have a decreased ability to reconstitute myelopoiesis after transplantation, but the mice have normal mature hematopoietic parameters and are otherwise healthy. It is therefore conceivable that patients would tolerate potent suppression of FLT3 signaling without significant on-target adverse effects.
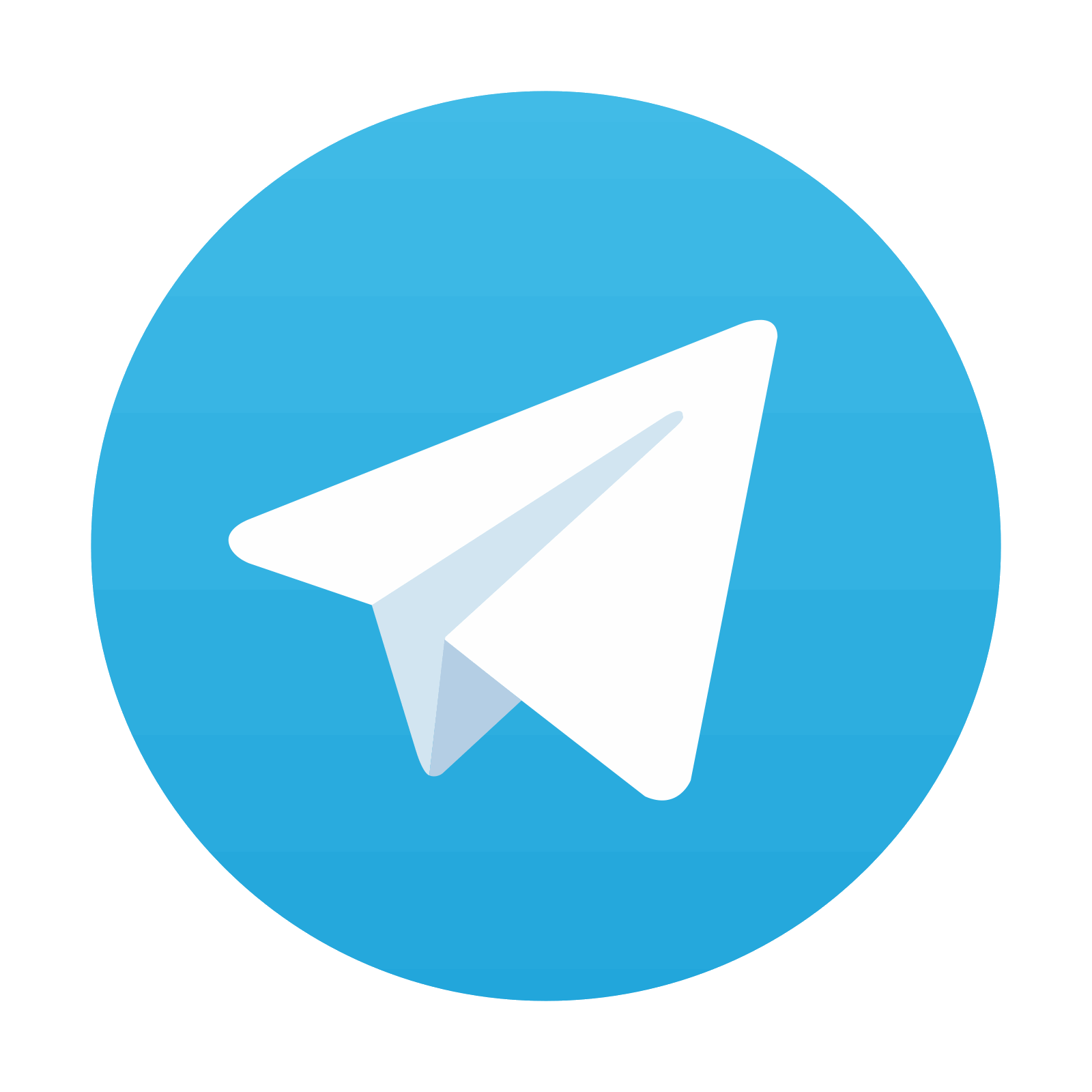
Stay updated, free articles. Join our Telegram channel
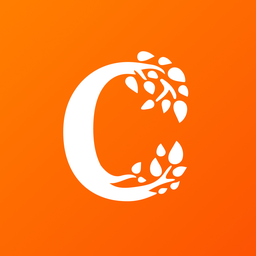
Full access? Get Clinical Tree
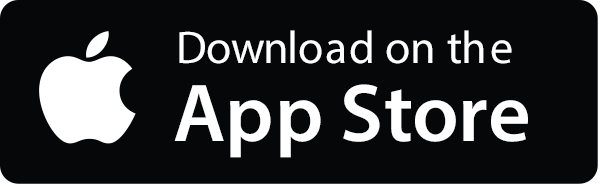
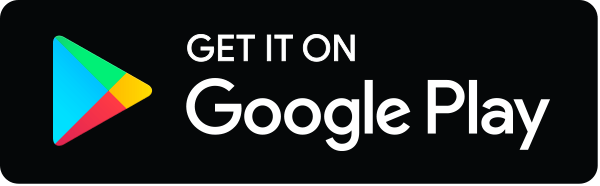