Keywords
siRNA, RNAi, cancer, targeted delivery, theranostics
Introduction
RNA interference (RNAi) is a relatively new and highly promising technology for gene therapy ( Figure 4.1 ). RNAi is a gene silencing process in which double-stranded RNA (dsRNA) interferes with the expression of a gene through a homologous sequence shared with the dsRNA . RNA silencing occurs when the RNase III enzyme Dicer initiates the cytoplasmic breakdown of dsRNA into small interfering RNA (siRNA) . These siRNA fragments, generally 20–25 nucleotides in length, incorporate into the RNA-induced silencing complex (RISC) . Once incorporated into the RISC, siRNA is unwound into a single-stranded RNA (ssRNA) where the sense strand ssRNA is then degraded . The RISC containing sequence-specific antisense strand of ssRNA is then able to seek out and target mRNA that is complementary to the antisense strand . Cleavage of mRNA occurs at nucleotide position 10 and 11 on the complementary antisense strand, relative to the 5′ end . To further amplify gene silencing, the RISC can continue on to destroy additional mRNA targets, allowing the therapeutic effect to last for up to 7 days in rapidly proliferating cells and for several weeks in nondividing cells .
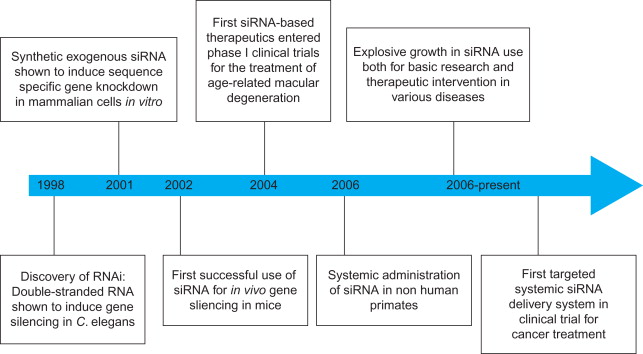
Although naturally generated from long dsRNA, siRNA can be synthetically produced to initiate RNAi. As demonstrated in Figure 4.2 , direct introduction of synthetic siRNA into cells can bypass the Dicer mechanisms to trigger gene silencing . siRNA molecules have already been shown to block specific expression of endogenous and heterologous genes in several mammalian cell lines. Furthermore, long-term gene silencing can be achieved without interrupting endogenous microRNA pathways through multiple administrations of synthetic siRNAs . siRNA’s high potential as a biopharmaceutical derives from its ability to induce RNAi, a mechanism that interferes only with translation and not with DNA transcription. Because siRNA does not interact with chromosomal DNA, the lack of DNA interaction reduces the possibility of adverse gene alterations found in DNA-based gene therapy. Through the appropriate design of siRNA, it is theoretically possible to use RNAi to silence nearly any gene in the body, providing a much broader therapeutic potential than conventional small-molecule drugs . It has been reported that synthetic siRNAs are able to knock down targets in various diseases in vivo , including hepatitis B virus, human papillomavirus, ovarian cancer, bone cancer, hypercholesterolemia, and liver cirrhosis . Moreover, only a few molecules of siRNA per cell are required to produce effective gene silencing .
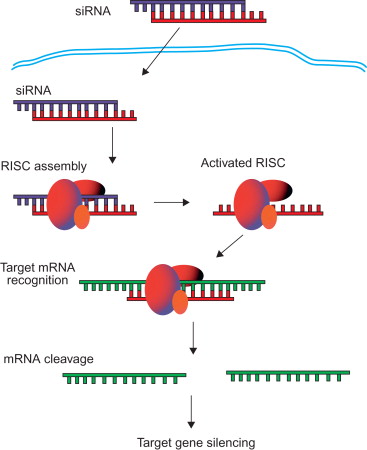
The discovery of RNAi and the subsequent use of siRNA to exploit this mechanism has revealed new opportunities for the development of novel therapeutic systems to treat previously incurable diseases. The ability of siRNA to target any gene with a complementary sequence makes it a potentially potent therapeutic, especially for cancer . During the past several decades, many important genes associated with different cancers have been identified along with their mutations and pathways through which they can be characterized . As a genetic disease, cancer is a well-suited candidate for siRNA-mediated gene therapy. Already, a number of siRNAs have been developed against dominant oncogenes, malfunctionally regulated oncogenes, and viral oncogenes involved in carcinogenesis. In addition, siRNAs have been studied as a therapeutic means of silencing target molecules crucial for tumor–host interactions and tumor resistance to chemo- or radiotherapy. The consequent silencing of these critical cancer-associated target proteins by siRNAs has led to significant apoptotic and/or antiproliferative effects .
Although RNAi holds great potential for cancer therapy, many issues remain to be resolved in order to develop this technology into a viable bedside treatment option. The main challenge for clinical translation of RNAi is the efficient delivery of siRNA therapeutics into target cells. Safe and efficient delivery systems are needed to protect siRNA from enzymatic degradation during the process of in vivo delivery and to effectively transfect target cells. Significant progress has been made on the design and development of safe and effective siRNA delivery systems. Recently reported siRNA delivery systems aimed at various cancers are summarized in Table 4.1 . This chapter discusses the concept of systemic siRNA delivery for cancer therapy and the design and recent development of siRNA delivery systems.
Delivery Systems | Property | Target Gene | Animal Model | Route |
---|---|---|---|---|
Liposomes | SNALP | HBV | HBV vector-based mouse | i.v. |
Cationic liposome | Bcl-2 | Liver metastasis mouse model | i.v. | |
Cationic liposome | Integrin αv | Prostate cancer xenograft | i.t. | |
Cationic liposome | CD 32 | Prostate cancer xenograft | i.v. | |
Cationic liposome | Bcl-2 | Prostate cancer xenograft | i.t. | |
Cationic cardiolipin liposome | Raf-1 | Prostate cancer xenograft | i.v. | |
Neutral liposomes (DOPC) | EphA2 | Ovarian cancer xenograft | i.v./i.p. | |
Neutral liposomes (DOPC) | FAK | Ovarian cancer xenograft | i.p. | |
Neutral liposomes (DOPC) | ADRB2 | Ovarian cancer xenograft | i.p. | |
Neutral liposomes (DOPC) | IL-8 | Ovarian cancer xenograft | i.p. | |
Cationic immunoliposome | Lung metastasis | i.v. | ||
Immunoliposome | Her-2 | Breast cancer xenograft | i.v. | |
Nanoparticles | CaCO 3 nanoparticle | VEGF | Gastric cancer xenograft | i.t. |
Chitosan-coated nanoparticle | RhoA | Breast cancer xenograft | i.v. | |
Folated lipid nanoparticle | Her-2 | Nasopharyngeal cancer xenograft | i.t. | |
Polymers | PEI | Her-2 | Ovarian cancer xenograft | i.p. |
PEI | PTN | Orthotopic glioblastoma | i.c. | |
Poly (ester amine) | Akt | Urethane-induced lung cancer | Inhalation | |
Others | Atelocollagen | HPV18 | Cervical cancer xenograft | i.t. |
Chemical modification | HBV | HBV vector-based mouse | i.v. | |
Carbon nanotube | TERT | Lewis lung tumor | i.t. | |
Cyclodextrin-containing polycation | EWS-FLII | Metastatic Ewing’s sarcoma | i.v. |
Delivery Systems for siRNA
Considerations for Systemic siRNA Delivery
In order to harness the efficacious therapeutic effects of RNAi, siRNA must first be efficiently delivered to target tissue and cells. Systemic siRNA delivery from the bloodstream to the cytoplasm of the target cells has to overcome numerous challenges in the delivery process ( Figure 4.3 ). In the body, naked siRNA is susceptible to degradation by endogenous enzymes. Its large size (~13 kDa) and negative charge prevent siRNA molecules from crossing cellular membranes . To address these issues, siRNA can be complexed into nanoparticles to protect it from degradation and to facilitate cellular uptake. Once injected, the siRNA nanoparticles must travel in the circulatory system and avoid kidney filtration, phagocytosis, aggregation with serum proteins, and enzymatic degradation . Phagocytic cells such as macrophages and monocytes pose a significant immunological barrier in both the bloodstream and the extracellular matrix of tissues. Responsible for removing foreign materials as protection against viral infection, phagocytes are also efficient at removing therapeutic agents from the body . Another barrier to systemic siRNA delivery in vivo is the transport of the delivery vehicle from the bloodstream across the vascular endothelial barrier. Generally, only molecules less than 5 nm in diameter will cross the capillary endothelium, and larger ones will remain in circulation until they are taken up by tissues or organs and cleared from the body. Some tissues, such as the liver, spleen, and, in certain cases, tumors, allow larger molecules up to 200 nm in diameter—the size of typical drug delivery nanocarriers—to pass .
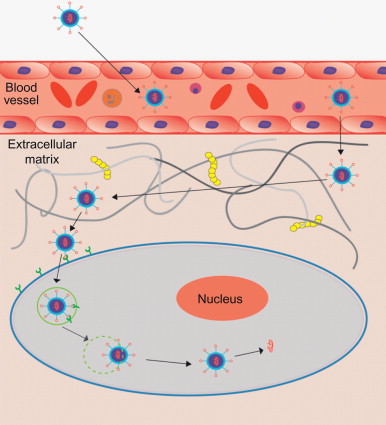
Once complexed siRNA exit the bloodstream, they must then diffuse through the extracellular matrix, creating further opportunity for uptake by phagocytic cells. The extracellular matrix is composed of a dense network of polysaccharides and fibrous proteins and creates a resistance against the transport of delivery vehicles that can interrupt the drug delivery process . Entry into the target cells involves endocytosis. Once the siRNA delivery systems have been taken up into the target cells, the next step in the delivery process is endosomal release, in which siRNA escapes from the endosomal compartments . If the siRNA complexes do not escape from the endosome, they will be trafficked through an endocytic pathway into lysosomes where siRNA can be degraded . To avoid this complication, delivery systems have been designed to disrupt the endosomal membrane and release the siRNA load into the cytosol. Cationic polymers swell and burst the endosome through protonation of excess amine groups through a process known as the “proton-sponge” effect . This mechanism is said to cause an influx of protons and chloride ions, leading to osmotic swelling and disruption of the endosome . Polyethyleneimine (PEI) was one of the first cationic polymers used for this and has proven to be an efficient delivery vehicle for siRNA. Finally, siRNA must be released from the carrier so that it can be incorporated into the RNA-induced silencing complex.
An ideal delivery system for siRNA should exhibit the following characteristics: (1) biocompatible (non-cytotoxic and nonimmunogenic), (2) biodegradable, (3) protect siRNA from enzymatic degradation, (4) minimal nonspecific tissue uptake, (5) tissue and cell specificity, and (6) efficient siRNA release in cytoplasm to allow interaction of siRNA with RISC.
Various delivery vehicles have recently been developed, providing the desired properties listed previously for the systemic in vivo delivery of siRNA ( Table 4.2 ). Delivery vehicles generally consist of cationic polymers or lipid-like materials and take advantage of the anionic nature of siRNA to form complexes through ionic interactions. These complexes provide protection against nuclease attack and facilitate cellular uptake of siRNA via endocytosis. Although the overall positive charges of the carrier–siRNA complexes improve cellular uptake due to favorable interaction with the negatively charged cellular membrane, many of the cationic carriers are cytotoxic, thus limiting their clinical potential . Various design strategies of delivery vehicles have been developed to improve properties such as stability, cellular delivery, and biocompatibility. Strategies ranging from the direct chemical modification of siRNA to different nonviral vehicles have been designed and evaluated. Various physicochemical and biological functions have been introduced to the delivery systems to protect siRNA from degradation, enhance cellular uptake, facilitate cytosolic siRNA release, and allow site-specific delivery. The development of biocompatible, efficient, and specific siRNA delivery systems to the target cancer cells is the key to translate siRNA into a bedside therapeutics.
Category of Particle | Type, Form, and Composition of Carrier | Natural versus Synthetic | Comments |
---|---|---|---|
Lipid-based | Cationic liposomes | Synthetic | Lung toxicity |
Neutral liposomes | Synthetic | Biodegradable–nontoxic | |
Lipoplexes | Synthetic | ||
Stable nucleic acid–lipid particles | Synthetic | ||
Cationic polymers | Chitosan | Natural | Biodegradable–nontoxic |
Atellocollagen | Natural | Biodegradable–nontoxic | |
PEGlyated | Synthetic | Cytotoxicity | |
Cyclodextrin | Synthetic | ||
Poly- l -lysine | Synthetic |
Systemic siRNA delivery is a convenient approach for cancer treatment. However, systemic delivery of naked siRNA experiences rapid renal clearance due to its small size . It has been shown that intravenous injection of naked siRNA results in its accumulation in the kidney and urinary bladder within 5 min of administration . Pharmacokinetics of siRNA can be altered by complexing it with carriers to form nanoparticles to prevent rapid clearance from circulation. Prolonged circulation of the siRNA delivery systems would allow preferential accumulation of the delivery system in solid tumor due to the enhanced permeability and retention (EPR) effect. The EPR effect is caused by the improper formation of the rapidly growing tumor vasculature, which causes it to be more permeable to large molecules and nanoparticles .
Systemic administration of nanoparticles often results in increased accumulation in the liver, spleen, kidneys, and lungs—the organs of the reticuloendothelial system (RES) . The surface property of a delivery system is a critical parameter for minimizing nonspecific tissue uptake and achieving target-specific siRNA delivery because it affects the interaction of the delivery system with the surrounding environment. A positively charged surface can readily associate with the negatively charged cellular membrane to facilitate uptake, including nonspecific uptake . However, negatively charged serum proteins in the blood plasma will complex with the positively charged nanoparticles, rendering the delivery systems ineffective. Hydrophilic biocompatible polyethylene glycol (PEG) chains are often used to modify the surface of the delivery systems to mask their positive surface and, consequently, to avoid aggregation in serum and to minimize nonspecific tissue and cellular uptake . PEG conjugation also plays an important role in protecting the delivery systems against the immune system and accompanying phagocytes. PEG forms a barrier around nanoparticles, creating steric stabilization and protection from the physiological surroundings . By altering the length of the PEG chain, the stabilization, protective properties, and particle size can be optimized for each delivery system . Targeting agents, mainly peptides and proteins, can be conjugated to the modified surface with a PEG spacer to achieve target-specific siRNA delivery.
Finally, the safety of siRNA delivery systems is the most important parameter to consider because even the most efficient delivery systems would be deemed useless if they elicit significant local or systemic toxicity. Early delivery vehicles studied, such as viral vectors, induced an immune response and thus were found to be toxic . Synthetic lipid and polymer systems have been developed to avoid stimulating an immune response. Although nonviral delivery systems do not induce significant immune reaction from the body, cationic carriers, especially cationic polymers, can cause serious toxic side effects after systemic administration. Biodegradable polycations containing environmentally sensitive linkages have been prepared to reduce the toxicity of the carriers . Surface modification of siRNA nanoparticles can also reduce the toxicity of the cationic carriers.
Targeted siRNA Delivery
Targeted siRNA delivery systems for cancer are a promising new class of experimental therapeutics with the potential to revolutionize medicine with their increased efficiency and relatively low toxicity compared to conventional drugs. The EPR effect allows siRNA nanoparticles to passively accumulate within tumors following system administration. However, because siRNA needs to be internalized by the cancer cells, the EPR effect alone may not provide the necessary means to ensure a therapeutic effect. Therefore, a strategy of active targeting to cancer cells is commonly used to enhance specific uptake in cancer cells. Active targeting involves the direct interaction of a targeting agent with a specific biomarker or receptor expressed on the surface of the target cells .
Various cell- and tissue-specific targeting agents, including various antibodies, peptides, or aptamers, have been identified and used for siRNA delivery . When selecting a targeting agent, it is important that the cellular receptor can be readily internalized after ligand binding and then re-expressed on the cell surface to allow repeated targeting. The selected targeting agent should also bind to its respective receptor with high specificity and affinity. Delivery vehicles equipped with targeting ligands are able to target and interact with specific cells to enhance cellular uptake via receptor-mediated endocytosis. Furthermore, because the majority of the chemical conjugations of these ligands occur on the carrier and not the nucleic acids of siRNA, the ability of siRNA for gene silencing will not be affected. Many surface modification techniques allow multiple ligands to be attached to the delivery vehicle. These multifunctional modification strategies combine ligands as a means of potentially overcoming sequential delivery barriers. The combination of targeting ligands and endosomal escape ligands can be used to elicit cell surface binding and receptor-mediated endocytosis and to promote delivery to the cytosol and avoid endosomal–lysosomal degradation, respectively . Cell-penetrating peptides are sometimes employed to enhance uptake, and they can increase cell membrane translocation of siRNA .
Studies have shown that some targeted siRNA delivery systems may not produce more favorable biodistribution or enhanced tumor uptake than nontargeted siRNA nanoparticles, but the former still result in more efficient gene silencing in cancer cells . For example, luciferase-specific DOTA-conjugated siRNA molecules were labeled with 64 Cu for positron emission tomography (PET). The labeled siRNA was then complexed with cyclodextrin-containing polycation nanoparticles both with and without transferrin (Tf) as a targeting ligand. The siRNA–nanoparticle complexes were administered to mice bearing tumors expressing luciferase. Figure 4.4A shows that PET imaging revealed that the attachment of the Tf targeting ligand to the surface of the nanoparticles had a negligible impact on the biodistribution compared to that of the nontargeted nanoparticles. Both Tf targeted and nontargeted nanoparticles were found to have similar tumor localization kinetics and similar tumor accumulation 1 day after injection. However, bioluminescent imaging (BLI) revealed that although tissue distribution for the targeted and nontargeted nanoparticles was similar, the targeted nanoparticles more effectively suppressed tumor luciferase expression 1 day after injection. The results revealed that a higher portion of the siRNA was able to localize in the target cells and thus actively functioned within the tumor cells when delivered using the Tf-targeted nanoparticles. Thus, the greatest advantage of using targeted delivery systems for siRNA is that targeted delivery systems are able to deliver more functional siRNA into tumor cells than nontargeted systems due to receptor-mediated endocytosis .
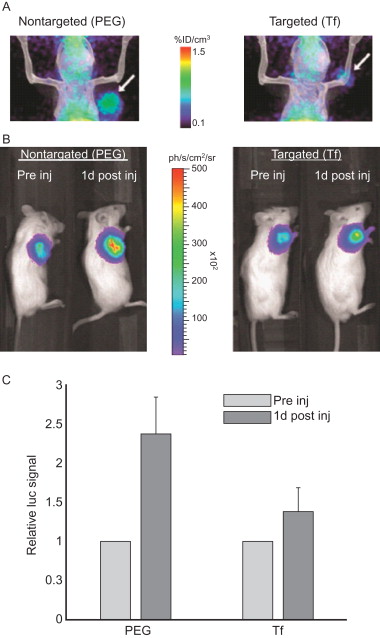
Systemic siRNA Delivery Systems for cancer Therapy
Chemically Modified siRNA for Cancer Therapy
siRNA can be chemically modified to alter its physicochemical properties and to increase its in vivo stability for improved circulation time ( Figure 4.5 ). Chemical modifications at various positions along the siRNA duplex have been made to both confer nuclease resistance and reduce nonspecific activation of the immune system . A common modification is the replacement of the phosphodiester group with a phosphothionate (PS) at the 3′ end . The introduction of phosphorothioate backbone linkages at the 3′ end of the RNA strands can inhibit enzymatic degradation by reducing siRNA’s susceptibility to exonucleases . The conversion of 2′-OH into an O -methyl group (2′- O -Me), a fluoro (2′-F) group, or a 2-methoxyethyl (2′- O -MOE) group leads to the decrease of hydrolysis associated with 2′-OH and an increase in half-life and RNAi activity in cells cultured with plasma . The modification of siRNA with 2,4-dinitrophenol (DNP) leads to improved nuclease resistance along with an increase in membrane permeability of the modified siRNA .
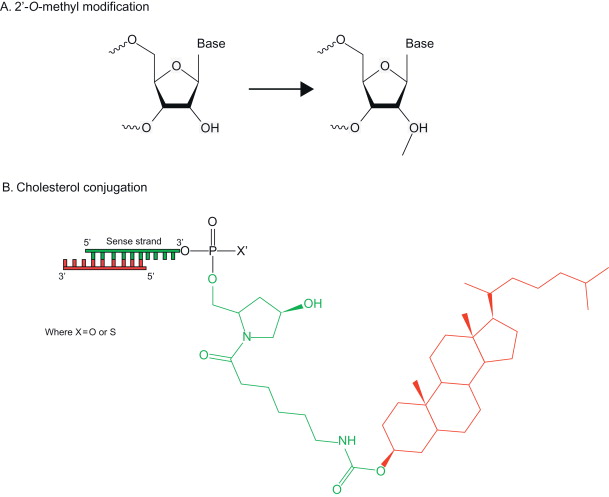
There may be concerns that direct siRNA modification may compromise gene silencing efficiency because RNAi evolves using native siRNA, and altered siRNA may impair its recognition by the RNAi machinery. Although it has been shown that siRNA modified with boranophosphate improved resistance to degradation, modification at the center position of the antisense strand reduced RNAi activity . The degradation of modified siRNA into molecules not found naturally in vivo may also raise concerns about introducing harmful by-products. As a result, focus has been placed on creating delivery systems in which unmodified siRNA will be loaded as a cargo for targeted systemic delivery.
Lipid-Based siRNA Delivery Systems for Cancer Therapy
Use of lipid-based transfection reagents is the most common approach for the in vitro delivery of nucleic acids to cells ( Figure 4.6 ). Liposomes have been developed for effective drug delivery and are also used for systemic siRNA delivery. Liposomes form spontaneously in aqueous environments when a lipid bilayer forms a sphere with an aqueous core. For example, a set of polar head groups can form the outer layer of the nanocomplex, whereas another set of polar head groups faces the interior hydrophilic core where the siRNA payload is held . Liposomes can also form an amorphous structure in which the lipids and nucleic acids of the siRNA are interspersed throughout. Liposomes can be easily tailored to have flexible physicochemical properties because multiple lipid types are incorporated in the lipid bilayer .
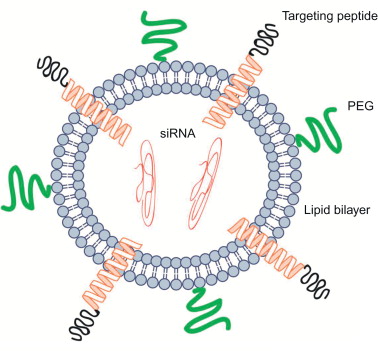
Several cationic lipid-based delivery systems have been developed for the in vivo delivery of siRNA for cancer therapy. Lipid composition, siRNA-to-lipid ratio, particle size, and assembly process are the key parameters to optimize when developing these delivery systems. Although cationic lipids are one of the most popular and effective nucleic acid delivery vehicles, there are concerns regarding their safety profile for therapeutic use . Both in vitro and in vivo toxicity have been reported for certain cationic lipid siRNA nanoparticles, and certain synthetic agents have been shown to increase off-target effects of siRNA . Consequently, recent interest has focused on developing nontoxic lipid delivery vehicles. Nevertheless, cationic lipids offer adequate protection against siRNA degradation by nucleases, improve cell membrane penetration, and reduce siRNA renal clearance, making them a promising siRNA delivery vehicle.
Cationic Liposomes
Liposomes have traditionally been a popular and widely used delivery system because of their good safety profiles and a superior payload compared to other delivery materials . Cationic liposomes have been developed for delivering nucleic acids, including DNA and RNA. Cationic lipids such as 1,2-dioleoyl-3-trimethylammonium propane (DOTAP) and N -[1-(2,3-dioleoyloxy)propyl]- N , N , N -trimethylammonium methyl sulfate (DOTMA), along with helper lipids such as DOPE, are often used to form cationic liposomes and complex with negatively charged DNA and siRNA, resulting in high in vitro transfection efficiency . Although efficient for in vitro nucleic acid delivery, cationic liposomes have had limited success for in vivo gene downregulation due to nonspecific tissue uptake and siRNA release. Cationic liposomes may also interact with serum proteins, lipoproteins, and the extracellular matrix, which cause premature release of the siRNA cargo. Furthermore, cationic liposomes can activate the complement system of the innate immune system, which causes the rapid clearance by activated macrophages of the RES. The surface of cationic liposomes can be modified with molecules such as PEG to minimize nonspecific tissue uptake and to enhance circulation half-life by eliminating aggregation and clearance by the RES. An extended circulation half-life allows for sustained availability to take advantage of the EPR effect, resulting in increased delivery to target sites. To promote cellular uptake, targeting agents can be incorporated to enable targeted delivery into a specific cell type. The combination of surface modification along with active targeting has been demonstrated with a PEGylated liposome formulation modified with antibodies against receptors for transferrin or insulin for targeted siRNA delivery to the brain in both mouse and monkey models .
Cationic immunoliposomal siRNA delivery nanoparticles modified with anti-transferrin receptor single-chain antibody fragment have been developed to silence human epidermal growth factor receptor-2 (HER-2) on human breast carcinoma cells. In a mouse xenograft model, repeat intravenous administrations of the immunoliposomes led to inhibition of HER-2 expression. The results of the in vivo delivery also demonstrated its ability to re-sensitize breast cancer cells to chemotherapeutic drugs . Another cationic liposome composed of dioctadecylamidoglycylspermidine (DOGS) has been used for gene silencing in breast cancer cells . The lipoplexes composed of the cationic liposomes complexed with the siRNA payload exhibited low cytotoxicity and mediated high uptake of cyclin D1-specific siRNA by MCF-7 breast cancer cells in the presence of serum. They accumulated within specific cytoplasmic compartments in the periphery of the nucleus. The cationic liposomes were also found to be effective delivery vehicles of siRNA specific for plasminogen activator inhibitor type 1 to breast carcinoma MDA MB 231 cells .
Neutral Liposomes
Neutral liposomes have also been used for systemic siRNA delivery. Neutral lipid 1,2-dioleoyl- sn -glycero-3-phosphatidylcholine (DOPC) forms liposomes approximately 65 nm in size and with a 65% siRNA encapsulation efficiency in DOPC-based neutral liposomes . The neutral liposomes containing anti-EphA2 siRNA have been tested for the treatment of ovarian cancer in animal models. The liposomes were administered intravenously in mice bearing human ovarian tumor xenografts. The liposomal EphA2-targeting siRNA inhibited ovarian tumor growth following treatment. When delivered in concert with paclitaxel, a significant reduction in the growth of ovarian cancers was observed in the mouse tumor model . The intraperitoneal administration of the liposomal EphA2-targeting siRNA was also evaluated and found to inhibit ovarian cancer growth by a similar degree compared to intravenous administration in the mouse tumor model. The DOPC-based neutral liposomes have additionally been used for the delivery of other anticancer siRNAs, including those targeting focal adhesion kinase, interleukin-8 (IL-8), and β 2 -adrenergic receptor, in ovarian cancer mouse models . The liposomes can deliver siRNA in vivo into tumors 10 and 30 times more effectively than cationic liposomes (DOTAP) and naked siRNA, respectively. Twice-weekly intravenous injections of DOPC liposomes at a dose of 150 μg/kg/day resulted in a substantial reduction in the expression of the target genes (e.g., EphA2, FAK, neuropilin-2, IL-8, or Bcl-2) and tumor size in mice with different human cancers, including subcutaneous xenografts and orthotopic tumor models . A single injection of DOPC liposomes (150 μg/kg, intravenously or intraperitoneally) leads to inhibition of target protein expression for more than 4 days in tumors in mice. DOPC-based liposomes did not cause any detectable distress or toxicity and were found to be safe in mice and in nonhuman primates. These liposomes do not exhibit any toxicity to normal cells, including fibroblasts, bone marrow, and hematopoietic cells, making them highly attractive for further development.
Lipid-Like siRNA Nanoparticles
In addition to liposomal formulations, lipid siRNA nanoparticles have been used in the systemic delivery of siRNA. Examples include stable nucleic acid–lipid particles (SNALPs) and cationic solid lipid nanoparticles (SLNs). SNALPs are constructed from a bilayer composed of a mixture of cationic and fusogenic lipids to enable cellular uptake and endosomal release of the siRNA cargo. They have been used to carry chemically modified siRNA targeting to HBV RNA as liver cancer therapeutics in mouse tumor models as well as to successfully deliver intravenously administered siRNA (2.5 mg/kg) into monkeys with marked inhibition of apolipoprotein B protein up to 11 days. siRNA delivered by SNALPs exhibited extended half-life in the liver and resulted in a 95% reduction in HBV serum titers. Delivery by SNALPs did not illicit an immune response, and the reduction in HBV DNA was sustained for up to 7 days following the last dose administration . SLNs reconstituted from natural components of protein-free low-density lipoproteins have also been used to deliver siRNA. SLNs were prepared using a modified solvent-emulsification method and composed of cholesteryl ester, triglyceride, cholesterol, dioleoyl phosphatidylethanolamine (DOPE), and 3-β-[ N -( N ′, N ′-dimethylamino ethane)carbamoyl]-cholesterol (DC-cholesterol). These carriers provide efficient target gene silencing and serum stability, with a minimal level of cytotoxicity.
pH-Sensitive Lipids
pH-sensitive cationic lipids have been developed as multifunctional carriers to form nanoparticles with siRNA and to promote endosomal escape of siRNA delivery systems. A multifunctional carrier (1-aminoethyl)iminobis[ N -(oleicylcysteinylhistinyl-1-aminoethyl)propionamide] (EHCO)) was developed and showed pH-sensitive amphiphilic cell membrane disruption at the endosomal pH ( Figure 4.7 ). It formed stable nanoparticles with siRNA, and the surface of the nanoparticles can be modified to reduce nonspecific tissue and cell uptake and to ensure targeted delivery. PEGylation of the siRNA/EHCO nanoparticles significantly reduced nonspecific cell uptake. The incorporation of a bombesin peptide or RGD peptide via a PEG spacer resulted in receptor-mediated cellular uptake and high gene silencing efficiency in U87 glioblastoma cells. Fluorescence confocal microscopic studies demonstrated that EHCO/siRNA nanoparticles and PEG-modified EHCO/siRNA nanoparticles facilitated endosomal escape of the siRNA delivery systems. Systemic administration of a therapeutic anti-HIF-1α siRNA with the peptide-targeted EHCO/ siRNA nanoparticles resulted in significant tumor growth inhibition compared to a nontargeted delivery system or free siRNA via intravenous injection in nude mice bearing human glioma U87 xenografts ( Figure 4.8 ). The results indicate great promise of the multifunctional carrier EHCO for systemic and targeted delivery of therapeutic siRNA to treat human diseases with RNAi .
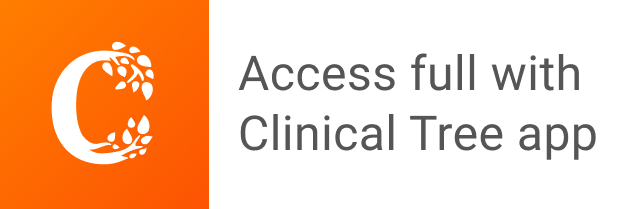