Surveillance method
Description
Advantages/benefits
Disadvantages/limitations
Thyroglobulin (Tg)
Protein product of thyroid follicular cells
Most sensitive method for detecting the presence of disease, particularly after stimulation with rhTSH or THW
Wide variability between assays
Measured in the serum by IMA, RIA, or LCMS. IMA is most common commercial method
Specific marker of thyroid follicular cells
TgAb interference occurs in up to 25% DTC patients, precluding reliable interpretation
Degree of elevation and rapidity of rise (doubling time) is a rough indication of disease burden particularly in differentiated disease
Does not localize disease
Neck ultrasound (neck US)
High-frequency sound waves are transformed into high-resolution 2-D digital images of the thyroid bed and cervical lymph node compartments
No radiation exposure
Operator dependent
Best method for detection of structural disease in cervical lymph nodes or thyroid bed
Clinical significance of small abnormal lymph nodes or residual tissue in thyroid bed is unclear, especially in low-risk patients with low or undetectable Tg
Easily coupled with FNA for suspicious lesions
Whole-body scan (WBS)
Nuclear medicine planar imaging is obtained after administration of an isotope of radioiodine (I-123 or I-131)
Functionally localizes iodine-avid areas of thyroid cancer or thyroid tissue
Less sensitive than serum thyroglobulin and neck ultrasound for low-level disease
Can be coupled with SPECT to correlate function and anatomy of areas of interest
Will not identify non-iodine-avid disease
Requires preparation with TSH stimulation and LID
Computed tomography (CT)
X-ray attenuation is used to calculate the value of pixelism, which becomes translated into an image
Anatomically localizes structural disease
Ionizing radiation exposure
Particularly useful for bulky or posterior neck disease or metastases to lung and mediastinum
Iodinated contrast exposure
Magnetic resonance imaging (MRI)
Body tissues that contain hydrogen atoms (e.g., in water) are made to emit a radio signal which are detected by the scanner. Search for “magnetic resonance” for physics details
Anatomically localizes structural disease
Expensive compared to CT
Particularly useful for bulky or posterior neck disease or metastases to brain, bone, and liver
Contraindicated in patients with metal implants or cardiac pacemakers
No ionizing radiation
Positron emission tomography (PET)
Nuclear medicine imaging is obtained after administration of radiolabeled glucose (18-FDG)
Can functionally localize radioiodine-avid and non-avid disease within or outside the neck
Blood glucose cutoff to perform the study may complicate testing in patients with uncontrolled diabetes or hyperglycemia
Useful for prognostication as well as disease detection
FDG-avid areas may not be thyroid cancer (risk of false positives)
Can be coupled with CT to correlate function and anatomy of areas of interest
Part II will describe the rationale for and potential harms of surveillance and the general approach based on initial risk stratification and subsequent response to initial therapy.
Part I: Surveillance Methods
Stimulated Testing
General Principles and Rationale
Surveillance for differentiated thyroid cancer can be performed under unstimulated or stimulated conditions. Unstimulated testing refers to testing that is performed while the patient continues to take thyroid hormone at replacement or TSH-suppressive doses. Hence, unstimulated testing is also known as testing “on suppression.” Stimulated testing refers to testing that is performed after thyroid hormone withdrawal (THW) for a time period sufficient to raise endogenous serum TSH > 30 mIU/L1 [17, 18] or after administration of exogenous recombinant human TSH (rhTSH or Thyrogen®) [19]. These methods of stimulation are known as withdrawal-based testing and rhTSH-based (or Thyrogen®-based) testing, respectively. The purpose of stimulated testing is to increase the sensitivity of the surveillance method in question, i.e., the likelihood of finding residual or recurrent disease.
Of the surveillance methods described below, whole-body iodine scanning is the only test performed solely under stimulated conditions. The sodium-iodine symporter responsible for uptake of iodine into thyroid follicular or epithelial cells is directly stimulated by TSH [20]. Residual thyroid cells—normal or cancerous—are therefore unlikely to take up radiolabeled iodine unless TSH is raised endogenously or administered exogenously. Moreover, it is also recommended that a period of dietary iodine restriction with low-iodine diet (LID) be undertaken prior to whole-body iodine scanning, again to facilitate uptake of radiolabeled iodine into any remaining thyroid cells. Data for LID is less robust than that for raising the TSH, but the potential for enhancing sensitivity of WBS is felt to outweigh the relatively limited harms (primarily inconvenience and unpalatable diet) of dietary iodine restriction [21].
Serum thyroglobulin can be measured on suppression or after stimulation. The sensitivity of thyroglobulin for detection of disease is greater with stimulation, since TSH stimulates the release of the thyroglobulin protein from thyroid epithelial or follicular cells into the bloodstream [4]. With the advent of “ultrasensitive” thyroglobulin assays, stimulated thyroglobulin testing can be deferred in many low-risk patients with no clinical or radiographic evidence of disease with undetectable thyroglobulin on suppression [22]. But for patients at intermediate or high risk for thyroid cancer recurrence, or those with a moderate to high pretest probability for recurrence, stimulated thyroglobulin measurement remains a powerful tool for detection [4].
Anatomic imaging studies—which include neck ultrasound and cross-sectional imaging (CT, MRI)—do not change based on serum TSH. Hence, there is no need to perform these tests under stimulated conditions. Positron emission tomography (PET) scanning, a functional imaging technique that visualizes uptake of a radiolabeled analog of glucose (18F-FDG) into areas with high metabolic activity, may demonstrate improved sensitivity under stimulated conditions. Elevated TSH is known to induce expression of glucose transporters in thyroid follicular cells, resulting in greater visualization on PET [23]. This effect, however, is not as pronounced as the effect of elevated TSH on the sodium-iodine symporter. Thus, raising serum TSH is not essential for PET, in contrast to the conditions required for whole-body iodine scanning [7]. The decision to recommend stimulation with THW or rhTSH prior to PET should be based on the individual clinical scenario. Patients who are already undergoing THW or rhTSH in preparation for stimulated thyroglobulin and WBS, and in whom PET is also needed, would not experience any additional logistical or preparation burden for a stimulated PET and might benefit from improved diagnostic yield. Likewise, patients in whom disease was previously detected on stimulated PET may need to be followed with repeat stimulated PET to adequately assess response to therapy on an analogous exam.
Figures 22.1 and 22.2 provide detailed timelines for preparation of DTC patients for stimulated testing with THW or rhTSH, respectively.



Fig. 22.1
Sample timeline for THW-based testing. *If TSH is <30, repeat in 3–5 days and defer dWBS until TSH is ≥30; also repeat Tg and TgAb when TSH is ≥30. Note: Steps 3, 5, and 6 can be omitted if only a stimulated Tg is desired, i.e., without dWBS. THW thyroid hormone withdrawal, RAI radioiodine, LID low-iodine diet, LT4 levothyroxine, LT3 liothyronine, dWBS diagnostic whole-body scan

Fig. 22.2
Sample timeline for rhTSH-based testing. Note: Steps 1, 4, and 5 can be omitted if only a stimulated Tg is desired, i.e., without dWBS. rhTSH recombinant human TSH, RAI radioiodine, LID low-iodine diet, dWBS diagnostic whole-body scan
THW Versus rhTSH
Evidence for THW in general, as well as for various withdrawal protocols, comes largely from patients being prepared for treatment with radioiodine rather than from patients being prepared specifically for diagnostic or surveillance testing. In early observational studies, endogenous elevation of TSH to >30 mIU/L appeared necessary for thyroid remnants to significantly concentrate administered radioiodine [17]. More recently, two randomized controlled trials reported that either directly stopping levothyroxine for 4–5 weeks or substituting levothyroxine with liothyronine for the initial 2–3 weeks resulted in similar short-term quality of life and hypothyroidism symptom scores; and in patients being prepared for treatment, rates of successful ablation were similar [18, 24]. One of the RCTs included patients being prepared for diagnostic scanning, but the number of patients was very small, and the ability to detect residual or recurrent disease or persistent thyroid tissue was not specifically studied [24].
The extremely favorable prognosis of most cases of DTC, coupled with the rapidly increasing incidence and detection of low-risk tumors since the early 1990s, intensified interest in surveillance protocols that were safe and better preserved quality of life. Specifically, there was a need for methods to raise serum TSH without inducing clinical hypothyroidism. Bovine TSH proved effective but was abandoned due to the development of allergic reactions as well as TSH-neutralizing antibodies [25]. Reduction of daily levothyroxine to half the usual dose was proposed but not confirmed in large series. Moreover, this approach attenuated but did not entirely avoid the consequences of clinical hypothyroidism [26].
The large-scale production of recombinant human TSH became possible after the β-subunit coding of the TSH gene was cloned, allowing for overexpression of the encoded TSH protein in a cell system [27–29]. (TSH is a pituitary glycoprotein composed of an α-subunit shared by the pituitary gonadotropins FSH and LH and a hormone-specific β-subunit.) Recombinant human TSH has the same biologic properties as native TSH and an identical amino acid sequence, but is less glycosylated and has more sialic acid. While these differences result in a lower affinity of rhTSH to the TSH receptor compared to endogenous TSH [27], preclinical in vitro and animal studies demonstrated that rhTSH increased serum T4 and T3 and stimulated radioiodine uptake into thyroid cells [30, 31]. Additional studies showed that rhTSH was a potent stimulus for T4, T3, and thyroglobulin release in normal human subjects [32, 33].
Initial clinical trials of rhTSH in DTC patients included several phase I/II studies for dose-finding and pharmacokinetics [34], followed by two phase III studies which established its safety and efficacy in this population [19, 35]. The first phase I/II study was completed in 1994 in 19 patients following thyroidectomy. It showed that rhTSH administration at doses of 0.9–3.6 mg for 1–3 days produced iodine scans of equal quality and with similar number of abnormal uptake sites in 63% of subjects. Serum thyroglobulin more than doubled in 73% after rhTSH, though the absolute rise in TSH was lower after rhTSH compared to THW in most subjects [34].
The first phase III study was conducted between 1992 and 1995 across multiple centers in the USA. One hundred twenty-seven thyroid cancer patients underwent two 131I whole-body scans, the first after rhTSH (0.9 mg IM for two consecutive days) while remaining on levothyroxine and the second after withdrawal from thyroid hormone. Compared to THW, preparation with rhTSH was associated with significantly fewer symptoms of hypothyroidism, but in 29% of patients was less sensitive for detecting residual or recurrent disease by 131I whole-body scan. The study may have been limited by the high percentage of negative scans (51% of patients) and scans with uptake in the thyroid bed only (72% of those with a positive scan), as well as by variation in the diagnostic dose of 131I and a lack of inclusion of serum thyroglobulin as an endpoint [35].
The second phase III study, performed across multiple centers in the USA and Europe, addressed some of these limitations. It was designed to compare two different dose regimens of rhTSH, as well as compare rhTSH-stimulated results to THW-based results. A total of 229 thyroid cancer patients were randomized; those in arm I received two injections of 0.9 mg on two consecutive days, and those in arm II received three injections of 0.9 mg 3 days apart. Serum thyroglobulin was included as an endpoint, both alone and in combination with WBS. The dose of 131I was fixed at 4 mCi and scanning procedures were standardized. Peak serum TSH concentrations occurred 24 h after the last rhTSH injection in both groups, though elevation of TSH persisted for longer in arm II than in arm I (9 days versus 4 days). Peak thyroglobulin levels occurred 3 days after the last rhTSH injection in arm I and 1–3 days after the last rhTSH injection in arm II. In both groups, the peak thyroglobulin was lower after rhTSH than after THW. Overall, 89% of patients had concordant scans, 16% had superior scans after THW, and 4% had superior scans after rhTSH. There was no difference between the two dosage regimens of rhTSH, suggesting the more convenient two-dose regimen could be used. The combination of serum thyroglobulin and WBS after rhTSH identified 100% of patients with metastatic disease and 93% with uptake in the thyroid bed. Moreover, a thyroglobulin cutoff of 2 ng/mL predicted thyroid bed uptake in 52% of patients and metastatic disease in 100%. Similar to prior studies, rhTSH preparation resulted in a much better quality of life, and it did so without causing significant side effects or anti-rhTSH antibodies [19].
On the basis of these studies, rhTSH was approved in the USA in 1998 and Europe in 2001 as a diagnostic tool in patients being tested for persistent or recurrent well-differentiated thyroid cancer [36]. Since its approval, multiple published studies have confirmed its efficacy and safety. As compared to THW-based regimens, rhTSH-based preparation has been associated with significant improvements in multiple quality of life measures [37, 38] and in some cases (particularly the elderly and those with underlying medical illness that are exacerbated by hypothyroidism) avoidance of the significant medical consequences of THW [39]. Another advantage of rhTSH compared to THW is the shorter duration of exposure of any remaining cancer cells to high levels of TSH, with potential to minimize the risk of residual tumor growth [40]. Indeed, suppression of TSH with supraphysiologic doses of thyroid hormone is one of the mainstays of long-term management of DTC. It thus follows that limiting exposure to elevated TSH levels might be beneficial.
Given similar rates of disease detection in most patients, and clearly improved quality of life with use of rhTSH compared to THW, under what circumstances might THW be selected instead of rhTSH to prepare a patient for stimulated surveillance studies? There have been several reports of acute swelling of known thyroid cancer lesions after rhTSH [41, 42], presumably due to the rapid surge in TSH that resulted in clinical compromise. Thus, THW might be considered if there is a strong suspicion for residual or recurrent disease in or near critical structures. Examples include metastases to the brain or near the spinal cord or skeletal metastases in weight-bearing bones susceptible to fracture. However, swelling at such sites, particularly in patients with a history of large-volume or bulky disease, is not unique to rhTSH stimulation. It is well known to occur after endogenous TSH stimulation with THW as well. Fortunately, serum thyroglobulin and imaging studies on levothyroxine suppression are usually already positive in patients with significant residual disease, or the clinical history and past treatment record indicate patients who are at higher risk. In such cases, administration of glucocorticoids prior to stimulation with rhTSH or THW may prevent or mitigate tumor expansion [43].
Financial or logistical circumstances may favor THW over rhTSH in select situations. On a societal level, the high cost of rhTSH may be balanced or offset by the decrease in morbidity and increase in productivity (e.g., fewer missed work days) associated with avoiding prolonged hypothyroidism. Several but not all studies examining this issue have concluded that rhTSH for these reasons represents good value for money, with the benefit to patients and society obtained at modest net cost [38, 44–46]. On an individual level, however, rhTSH is not affordable to everyone. rhTSH is expensive, with costs and insurance reimbursement rates varying widely among different countries. In the USA, for example, patients without insurance or those with high out-of-pocket costs of medication may be unable to afford rhTSH injections [45].
From a logistical perspective, the extra clinic or hospital visits required for rhTSH-based preparation may not be feasible, particularly if the patient’s primary residence is located far from the site of rhTSH administration. Patients, who tolerate THW well but cannot attend four visits within a single week for rhTSH injections, WBS, and thyroglobulin measurement, may opt for THW since the scan and stimulated thyroglobulin can be obtained with only two visits [8]. Finally, while not a reason in itself to select THW over rhTSH, it should be noted that rhTSH-based surveillance is more time sensitive than THW-based withdrawal. Diagnostic dose of 131I should be given 24 h after the second rhTSH injection (with images obtained 48 h after the 131I), and measurement of stimulated thyroglobulin should be done approximately 72 h after the second rhTSH injection [8]. If the patient misses any of these critical appointment times, rhTSH must be readministered (which may not be feasible due to cost) before obtaining WBS and stimulated thyroglobulin. By contrast, patients withdrawn from thyroid hormone may undergo WBS and serum thyroglobulin measurement any time after the serum TSH reaches >30 mIU/L, allowing for greater flexibility in case of unanticipated delays. Similarly, if there is a high suspicion for residual disease necessitating a therapeutic dose of 131I, preparation with THW may be preferred, since the therapeutic dose could be administered soon after the diagnostic WBS and thyroglobulin results are available. For patients prepared with rhTSH, an additional two injections of rhTSH (or subsequent THW) would be needed to treat any disease detected on surveillance testing.
Overall, rhTSH-based preparation for stimulated surveillance studies is preferred over THW in most situations. A good understanding of the specific advantages and limitations to each approach allows for identification of patients who may benefit (or are unlikely to suffer significant harm) from THW, thus facilitating a patient-centered approach that includes informed decision making.
Low-Iodine Diet
It is recommended that patients follow a low-iodine diet (LID) prior to whole-body radioiodine scanning for treatment or diagnostic purposes, to facilitate increased uptake of radioiodine into any remaining thyroid cells. This recommendation is based primarily on observational studies of patients who followed an LID in preparation for remnant ablation or treatment with 131I [21]. No studies have specifically examined the efficacy or safety of LID in patients undergoing surveillance WBS.
Of 8 studies examined in a systematic review, in most cases, patients were restricted to < 50 mcg of dietary iodine per day for 1–2 weeks, though the duration of the LID varied from 4 days to 4 weeks. All LIDs studied were associated with significantly lower urinary iodine excretion, as well as increase in 131I uptake into residual thyroid or tumor, compared to diets free of iodine restriction [21]. In two of the studies included in the review, using an LID for 2 weeks resulted in an approximate 50% reduction in mean urinary excretion compared to the same diet for 1 week [47, 48]. (A subsequent study published after the review paper showed that a 3-week LID did not result in any further reduction in mean urinary excretion compared to a 2-week LID [49].) Only one study in the review paper examined LID in combination with rhTSH administration; the remainder used LID in conjunction with THW [21, 47]. While urinary iodine excretion significantly decreased after 2-week LID with rhTSH, the reduction was not as robust as the same 2-week LID with THW [47]. The addition of diuretic therapy to LID did not appear to further lower urinary iodine measurements in the one study that measured urinary iodine with and without concurrent ethacrynic acid [50].
No investigators have specifically studied whether using an LID for remnant ablation or treatment improves long-term disease recurrence or mortality. There is some evidence that remnant ablation is more successful with LID, but data is conflicting and for the most part derived from retrospective analyses that used historical controls [51, 52]. In terms of safety, only one study included in the systematic review reported on side effects of LID, noting that the only complaint from participants was the “boring” nature of the diet [53]. No other adverse effects were noted. However, multiple cases of severe, potentially life-threatening hyponatremia following an LID have been reported [54, 55]. Most such cases involve elderly patients who were withdrawn from thyroid hormone, frequently in the presence of metastases to the lung or brain and in some instances treated simultaneously with thiazide diuretics. Duration of diet was also greater than 1 week in the majority of cases where hyponatremia developed [56].
In summary, despite the inconvenience of LIDs, the demonstrated reduction in urinary iodine excretion and increase in radioiodine uptake supports their use for 1–2 weeks prior to surveillance WBS. For elderly patients, particularly those with known thyroid cancer metastases, measures should be undertaken to minimize the risk of dangerous hyponatremia, including avoidance of co-administered diuretics, possible limitation of LID to 1 week, and consideration of stimulation with rhTSH instead of THW. It is also important to communicate to patients that “low-iodine” diet does not imply a “low-sodium” diet; non-iodized salt is permitted and can mitigate the risk of hyponatremia. Descriptions of low-iodine diets and general instructions can be found at several websites, including that of the American Thyroid Association (http://www.thyroid.org/low-iodine-diet/) and the Thyroid Cancer Survivors’ Association (http://www.thyca.org/pap-fol/lowiodinediet/).
Finally, ascertaining exposure to high amounts of iodine in the weeks to months preceding WBS—through vitamins, dietary supplements, or iodinated contrast medium including that given during contrast-enhanced CT scan or cardiac catheterization—is particularly important. If such exposures have occurred, an LID for a few weeks will be insufficient for adequate radioiodine uptake. The WBS would need to be delayed until the exogenous iodine load has been excreted, which could be assessed by serial measurements of urinary iodine excretion or waiting at least 4–6 weeks. Although studies of patients with intact thyroid glands have shown that total body iodine stores are increased for several months following iodinated contrast exposure, a study conducted in thyroid cancer patients following thyroidectomy demonstrated that the urinary iodine excretion returned to normal by 4 weeks [57].
Serum Thyroglobulin
Thyroglobulin is the precursor glycoprotein from which thyroid hormone is synthesized, following iodination of its tyrosine residues and coupling of mono- and diiodotyrosines. It is co-secreted into the circulation from the normal thyroid epithelial cells along with thyroid hormone. It is also produced by almost all differentiated thyroid cancer, but not by other tissues or cancers. Thus, a detectable thyroglobulin indicates the presence of functioning thyroid epithelial cells, either from normal thyroid tissue or from a follicular cell-derived thyroid cancer. Its absence is generally reassuring for DTC remission, cure, or stability, depending on the clinical risk status of the patient [20].
There are several reasons why serum thyroglobulin has emerged as the most powerful tool in the long-term follow-up and surveillance of differentiated thyroid cancer. It is the most sensitive method for detection of disease, particularly when measured after stimulation with rhTSH or THW; it retains high specificity in patients who have undergone thyroidectomy and remnant ablation; and it correlates with the extent of tumor or disease burden in many cases [58].
Most thyroglobulin assays currently in use are “second-generation” assays that have functional sensitivities of ≤0.1 ng/mL, compared to earlier “first-generation” assays with functional sensitivities of 1 ng/mL [5]. Multiple studies have shown that a single stimulated thyroglobulin of <0.5–1.0 ng/mL in the absence of interfering antibodies carries a high likelihood—approximately 98–99.5%—of remaining disease-free on long-term follow-up [59–61]. By contrast, a stimulated thyroglobulin of >2 ng/mL is highly sensitive for identifying individuals with persistent disease [60]. Low-level stimulated thyroglobulin levels, i.e., those that are detectable but between 0.2 and 2 ng/mL, are generally followed over time if there is no clinical or radiographic evidence of disease. In such cases, the overall risk status of the patient and the trend in the thyroglobulin over time are important factors in determining the frequency and intensity of subsequent follow-up and the threshold for additional treatment [59, 62]. Progressive increases in thyroglobulin or short doubling time suggests that the remaining disease is likely to become clinically apparent [63, 64].
Very low or undetectable thyroglobulin values on suppression in assays with functional sensitivities of 0.1–0.2 ng/mL may obviate the need to obtain stimulated thyroglobulin values [65–67]. Suppressed thyroglobulin of <0.1 ng/mL in one study using a sensitive assay was associated with an rhTSH-stimulated thyroglobulin of >2 ng/mL in only 2% of cases [22]. Minimally detectable thyroglobulin values on suppression—in the range of 0.2–0.3 ng/mL—are associated with a higher but still overall low risk of stimulated thyroglobulin rising to >2 ng/mL. More importantly, the chance of finding clinically relevant disease in such patients is very low [65, 68]. How then are we to utilize modern-day assays that detect extremely low levels of circulating thyroglobulin and still preserve enough specificity to avoid unnecessary subsequent testing and possibly even treatment? Receiver operator curves have demonstrated that thyroglobulin levels on suppression of 0.2–0.3 ng/mL are associated with an optimal balance of sensitivity and specificity for detecting persistent disease [69]. Thus, patients with suppressed thyroglobulin levels in this range, negative thyroglobulin antibodies, and low clinical suspicion of disease may be followed without stimulated testing. For higher levels of thyroglobulin on suppression, in the range of 0.3–1 ng/mL, stimulated testing is still recommended since 20% will have a stimulated thyroglobulin of >2 ng/mL, and approximately one-third of these patients will be found to have persistent or recurrent disease [70].
Beyond its primary role in disease detection, thyroglobulin may also be useful for estimating residual disease burden in some patients. It has been estimated that 1 g of normal or differentiated neoplastic thyroid tissue increases the serum thyroglobulin by ~0.5 ng/mL when TSH is suppressed to <0.1, by ~1 ng/mL when TSH is normal, and by 2–10 ng/mL after rhTSH stimulation [71, 72]. This relationship has been studied primarily in individuals with clinically significant, macroscopic disease and in particular those with metastases. It is uncertain to what degree low levels of residual or persistent disease, e.g., microscopic disease in the cervical lymph nodes, will quantitatively raise thyroglobulin.
There are several important limitations to the use of serum thyroglobulin in the long-term follow-up of thyroid cancer patients. One is the continued variance in thyroglobulin assays among different laboratories, resulting in significant differences in thyroglobulin values measured on the same sample. Substantial variance can be seen regardless of type of assay used, including immunometric assay (IMA, the most commonly used), radioimmunoassay (RIA, less widely available, and perhaps less sensitive with low-level disease), or more recently liquid chromatography-tandem mass spectrometry (LCMS, least used at present) [5, 73, 74]. To limit potential variance, it is recommended that IMAs and RIAs be calibrated against the CRM-457 international standard. However, even with standardization, assays from different laboratories can differ by up to twofold, presumably due to the heterogeneity of the thyroglobulin protein and differences in the epitopes targeted by each assay. The clinical implication of this variation is that serum thyroglobulin levels in an individual patient should be measured longitudinally in the same laboratory whenever possible [5]. If serum thyroglobulin concentration must be measured in a different laboratory, for example, due to institutional change in methodology or patient relocation to a new care provider whose laboratory uses a different assay, the initial thyroglobulin level measured on the new assay should be treated as the patient’s new baseline. Any discordance from prior measurements should not be taken as absolute evidence of a true change in clinical status, but rather interpreted in light of the entire clinical picture.
The most challenging limitation encountered in serum thyroglobulin testing is the presence of antithyroglobulin autoantibodies (TgAbs), which are detectable in approximately 10% of the normal population [75] and around 25% of patients with differentiated thyroid cancer [76, 77]. These autoantibodies can cause false-negative or false-positive thyroglobulin results, depending on assay used, making thyroglobulin an unreliable marker of thyroid cancer in patients who harbor them. It is unclear why the prevalence of these antibodies is higher in patients with thyroid cancer than in normal individuals. Individuals with history of autoimmune thyroid disease, or with the background of Hashimoto’s thyroiditis on surgical pathology, are more likely to be TgAb positive [78]. In addition to TgAb, heterophile antibodies (primarily human anti-mouse antibodies or HAMAs) can also interfere with thyroglobulin measurement. The prevalence of HAMA is much less common (~ 0.5%) in thyroid cancer patients compared to TgAb and when present tends to falsely elevate the measured thyroglobulin level on immunometric assays. HAMA is not routinely measured but can be ordered if there is clinical suspicion, for example, if a low-risk, TgAb-negative patient with DTC and previously negative thyroglobulin is found to have a newly elevated serum thyroglobulin without any other evidence of recurrence [5, 79].
Unfortunately, there is no method that entirely overcomes interference from TgAb or HAMA. IMA is the assay type that is most affected. In the presence of TgAb, the thyroglobulin level on an IMA is usually falsely undetectable or low [80]. Thus, an “undetectable” thyroglobulin by IMA in a TgAb-positive patient cannot be interpreted as absence of disease. A detectable thyroglobulin level on IMA generally indicates that thyroglobulin is present, but its true concentration may be underestimated. Evidence for the use of “recovery assays” to detect potential interference in TgAb-positive patients is conflicting, so their use is generally discouraged [79, 80]. RIAs are less prone to interference from TgAb, but can still be affected. Unlike IMAs, however, RIAs usually produce falsely elevated thyroglobulin results if they are affected by TgAb [81–83]. Detectable thyroglobulin on RIA in a TgAb-positive patient therefore should not be used as the sole factor for determining the presence of residual thyroid tissue or tumor.
Most recently, an LCMS assay for thyroglobulin has been developed and may be able to accurately measure thyroglobulin even in the presence of thyroglobulin antibodies. Longer-term data regarding these assays’ sensitivities, correlations with immunoassays, and relationship to disease remission or persistence will help determine their role in the follow-up of differentiated thyroid cancer [84].
In the meantime, following the trend (i.e., the concentration) of thyroglobulin antibodies over time in patients who are antibody positive is useful. Thyroglobulin antibodies should be quantified using the same laboratory assay whenever possible because there is substantial variation in their measurement among different assays, just as occurs with measurement of thyroglobulin itself [79]. Progressively decreasing levels of thyroglobulin antibodies may indicate disease remission, or at least a low or declining tumor burden. Thyroglobulin antibodies usually become undetectable at a median of about 3 years in initially antibody-positive patients who remain disease-free [85, 86]. By contrast, increasing thyroglobulin antibody levels, or the emergence of thyroglobulin antibodies in a patient who was previously thyroglobulin antibody negative, raises concern for recurrent disease.
A final limitation to the use of thyroglobulin is its low production by some thyroid cancers, often but not always those with more aggressive histology or those that have become dedifferentiated. Sometimes low tumoral thyroglobulin production can be predicted preoperatively, for example, if a patient with known thyroid cancer has an unexpectedly normal or low-normal serum thyroglobulin even before thyroidectomy [72]. In other instances, poor thyroglobulin production by remaining tumor is suspected based on clinical or radiographic grounds, for example, if residual tumor is detectable on an imaging study in the absence of significantly elevated thyroglobulin. Patients whose cancers are “poor thyroglobulin producers” require more frequent surveillance by imaging studies.
Neck Ultrasound
The cervical lymph nodes are the most common site of spread outside the thyroid gland for papillary thyroid cancer. The majority of patients with PTC have cervical node involvement at the time of diagnosis, though in many cases these are not apparent preoperatively or at the time of initial surgery and often do not change the overall patient prognosis. This is particularly true of nodal micrometastases (those <2 mm) [87–89]. Likewise, ~90% of all recurrences of PTC occur in the cervical lymph nodes; and cervical lymph node recurrences have been reported in ~30% of patients. The central neck lymph nodes are more commonly involved than those in the lateral neck, but both central and lateral neck lymph node involvements are far more common than distant metastases in PTC [90]. The prevalence of cervical lymph node metastases is much lower in follicular thyroid cancer, on the order of 10%, and more commonly with the Hürthle cell subtype of FTC [91]. As papillary cancer comprises the great majority of DTC, an imaging modality that accurately identifies central and lateral lymph node metastases is essential in the surveillance of thyroid cancer patients.
Cervical ultrasonography has emerged as the most sensitive imaging method for detecting cervical lymph node metastases. Indeed, it has become the primary structural imaging modality for patients with differentiated thyroid cancer [13, 15, 16]. Using a high-resolution probe, with frequency of ≥10 MHz, abnormal lymph nodes as small as 2–3 mm can be detected [6]. No specific patient preparation in terms of diet and laboratory values is needed. Additional advantages compared to other imaging techniques include its noninvasive nature, lack of associated exposure to radiation, and permitted use in patient populations in whom radiation-emitting or contrast-based studies may be contraindicated (including pregnant and breastfeeding patients or those with advanced kidney disease). Moreover, it is the only imaging modality that can readily be coupled with fine-needle aspiration of any identified suspicious lesions [92].
If abnormal small cervical lymph nodes <5–7 mm in short axis are detected, close observation is generally recommended, as such lymph nodes may remain stable for long periods of time [93, 94]. Further, surgical resection may fail to produce a biochemical remission in up to 73% of patients [95]. If abnormal cervical lymph nodes measuring ≥8–10 mm in size are detected, fine-needle aspiration is general recommended, assuming that confirmation of disease would lead to change in management. In 80% of cases of cervical lymph node metastases, cytology is confirmatory. The diagnostic yield is improved when measurement of thyroglobulin in the aspirate fluid (Tg-FNA) is performed in addition to cytology [96, 97]. Tg-FNA samples are typically obtained by washing 1 mL of normal saline through the needle used for fine-needle aspiration into a sterile plain tube or container, after the contents of the biopsy needle have been placed onto a slide for cytology. The ability to measure thyroglobulin in the aspirate fluid is especially advantageous in patients with circulating thyroglobulin antibodies. Unlike serum thyroglobulin measurement, the measurement of thyroglobulin in cervical lymph node fluid is not affected by circulating thyroglobulin antibodies [98]. A thyroglobulin level of 1–10 ng/mL in aspirate fluid is considered suspicious for malignancy, and a value of >10 ng/mL is highly likely to represent DTC that has spread to the aspirated lymph node [99, 100].
Features concerning for recurrence in the thyroid bed include ovoid shape of a lesion in the longitudinal plane (and taller than wide in the transverse plane), hypoechogenicity, microcalcifications, irregular borders, and increased vascularization [101]. Features most concerning for cervical lymph node metastases include cystic appearance, microcalcifications (hyperechoic punctuations), and peripheral vascularization [15]. These lymph node findings have specificities in the range of 80–100%, thereby justifying fine-needle aspiration when present individually or in combination in a lymph node measuring 8 mm or larger [102]. Lymph nodes that have lost their normal hyperechoic fatty hilum, and those that are round in shape or hypoechoic, are considered suspicious, but in the absence of other concerning features are less specific for malignancy. Thus any one of these latter findings, if present in isolation, does not automatically warrant fine-needle aspiration [13, 15, 103].
It is critical that abnormalities on neck ultrasound be interpreted in the context of the patient’s clinical picture and pretest probability of disease. In low- and intermediate-risk patients with an undetectable serum thyroglobulin, the risk of lymph node recurrence is <2% [15]. Minor abnormalities are more likely to represent false-positive findings than true disease in this population, and even in the case of true disease, the clinical significance of small recurrences <8–10 mm is unclear [104]. A substantial portion of patients in this category may experience stability or regression of the abnormal cervical nodes over time, bringing into question the benefit of intervention, and supporting a strategy of continued observation. On the other hand, abnormal lymph nodes in patients with detectable or elevated thyroglobulin are more likely to grow or become clinically significant over time, warranting at minimum attempts at diagnostic aspiration [105].
The main limitation of cervical ultrasound is its dependence on the skill of the operator. A thorough ultrasound exam must include evaluation of the thyroid bed as well as the central and lateral cervical lymph node compartments. Individuals performing ultrasound should be specifically trained and experienced in thyroid disease and pathology, including imaging of the postoperative neck, in order to approximate the high sensitivity of neck ultrasound reported in the literature [92]. Traditionally, dedicated sonographers within radiology departments performed most neck ultrasounds. However, with the rising use of neck ultrasound in thyroid cancer surveillance, and the growing recognition that abnormal findings may be best interpreted in the context of the patient’s entire clinical picture, endocrinologists and surgeons who care for thyroid cancer patients are increasingly performing their own office-based neck ultrasounds. Office-based ultrasound also allows for “real-time” manipulation of the probe, with improved ability to identify abnormalities that are concerning for recurrence and distinguish them from less concerning findings such as postoperative scar or suture-related granuloma [106]. If abnormal findings are reported from a center with less thyroid disease experience, or normal findings are reported in the setting of a high clinical suspicion for recurrence, the images should be reviewed by a center or provider with specific training in thyroid cancer and a high volume of DTC patients.
Whole-Body Scanning
Iodine-131 has occupied a central diagnostic and therapeutic role in the management of patients with differentiated thyroid cancer since its discovery in 1938. It is taken up by normal thyroid as well as most differentiated thyroid cancer cells, emitting ~10% of its energy and dosage via gamma radiation that can be visualized by gamma camera. [The other 90% of its dosage undergoes beta decay, which does not contribute to visualization but does cause destruction of immediately surrounding tissue at higher doses.] Another radioisotope of iodine, 123I, emits primarily gamma radiation and is thus used for diagnostic but not treatment purposes. It has a shorter half-life and may produce superior images, but since it is more difficult to create compared to 131I, it is also more expensive [107]. Regardless of the isotope used, diagnostic doses are usually in the range of 2–5 mCi, and diagnostic whole-body scan to visualize remaining thyroid tissue in the thyroid bed, neck, or distant sites is performed approximately 24 h (for 123I) or 48 h (for 131I) later. Planar images are produced [58].
The main advantage of dWBS as a functional imaging technique in the surveillance of DTC is its potential to identify the location of any remaining thyroid tissue, including neoplastic tissue, throughout the body. It is especially useful in determining whether known or suspected persistent or recurrent disease is radioiodine avid, allowing for prognostication and treatment planning [58]. When planar imaging is combined with SPECT/CT, functional and anatomic imaging can be superimposed, significantly increasing both the sensitivity and the specificity of the dWBS. Normal thyroid remnants may be better differentiated from lymph node metastases due to the presence of anatomic landmarks on SPECT/CT. Similarly, SPECT/CT can help determine lung versus rib uptake, as well as physiologic accumulation of 131I in the gastrointestinal tract or bladder versus pathologic pelvic lesions [108, 109].
On the other hand, dWBS remains significantly less sensitive compared to serum thyroglobulin or cervical neck ultrasound, in particular for the detection of low-level disease [110–112]. Current evidence suggests that low-risk patients with no uptake outside the thyroid bed on posttreatment WBS (at 1 week after initial radioiodine treatment or remnant ablation), undetectable serum thyroglobulin and thyroglobulin antibodies while on thyroid hormone, and negative neck ultrasounds can forgo dWBS at the time of subsequent surveillance testing, since it is unlikely to yield additional useful information. Diagnostic WBS is usually still indicated in the follow-up of patients at intermediate or high risk for recurrence and for low-risk patients who do not fulfill the above criteria. Patients with large thyroid remnants, generally those demonstrating >2% uptake of the administered radioiodine dose, may also benefit from dWBS at the time of stimulated surveillance testing, since residual thyroid tissue can obscure visualization of cervical lymph node disease [113, 114].
Disadvantages of dWBS, in addition to its low sensitivity compared to serum thyroglobulin and cervical neck ultrasound, include the need for pre-procedure preparation with TSH stimulation by either thyroid hormone withdrawal or rhTSH stimulation, as well as with LID. The process of TSH stimulation carries risks of hypothyroid symptoms (for THW) and possible growth or swelling of remaining thyroid cancer (for either THW or rhTSH) [41, 115]. Both the TSH stimulation and LID components of preparation can be logistically challenging for patients. Further, patients are also exposed to radiation with dWBS. While the exposure is far less compared to therapeutic doses of radioiodine, the doses of 131I administered for dWBS may cause “stunning” of remaining thyroid tissue, rendering these foci less amenable to uptake of future therapeutic doses of 131I. When there is a concern for possible “stunning,” 123I can be used in place of 131I for dWBS [116, 117]. Finally, aggressive or dedifferentiated forms of thyroid cancer—precisely the forms which are the most important to detect and treat—often lose their ability to concentrate iodine and are at high risk of being missed on dWBS. In some of these cases, an empiric high (or therapeutic) dose of 131I can lead to detection of thyroid cancer location on the 1-week posttreatment WBS, but the clinical benefit to patients is unclear [118, 119].
CT and MRI
Since neck ultrasound coupled with serum thyroglobulin will detect most cases of persistent or recurrent disease, cross-sectional imaging with computed tomography (CT) or magnetic resonance imaging (MRI) is not necessary in the standard follow-up of most patients with differentiated thyroid cancer. These tests do, however, play important roles in several situations. CT scanning of the chest and neck is recommended when thyroglobulin is rising and neck ultrasound is negative, particularly in high-risk patients or when thyroglobulin exceeds 10 ng/mL [13]. Helical or spiral CT of the chest is the most sensitive test for detecting lung metastases, as it can detect micrometastases in the lung as small as 2 mm. It can be performed without contrast for parenchymal lesions. The addition of contrast allows for assessment of the mediastinum as well as the neck [120, 121]. In cases of known or suspected bulky neck disease, CT of the neck with contrast can complement neck ultrasound, as it can better delineate the extent of disease behind the trachea and in the mediastinum [120, 122]. Moreover, if neck CT was performed at baseline for a patient with bulky or posterior lymph node involvement at presentation, CT should be a part of the initial surveillance strategy, so as to allow direct comparison to prior images and follow response of specific disease foci to treatment.
MRI is also suitable for imaging of the neck and mediastinum and may be preferred over CT when there is concern about iodinated contrast administration [123–125]. Providers may seek to avoid iodinate contrast (1) when radioiodine treatment or diagnostic whole-body scanning is anticipated in the near future, as either would need to be deferred for at least 4 weeks after a contrast CT study [57], or (2) if preexisting renal dysfunction predisposes a patient to iodinated contrast-mediated kidney injury. MRI may also be preferred when aerodigestive tract disease is a possibility, as some studies indicate it is superior to CT scanning in this situation [126, 127].
If no abnormality is found in the neck or chest (the first and second most common sites of DTC spread, respectively) to explain a rising thyroglobulin, again focusing on high-risk patients or patients in whom thyroglobulin exceeds 10 ng/mL, MRI of the brain, MR skeletal survey, and/or MRI or CT of the abdomen should all be considered to localize the site of thyroglobulin production [20]. MRI is the most sensitive tool for detecting liver lesions and also for differentiating metastases from benign entities such as angiomas, cysts, or benign nodules [128].
There is no clear-cut rule for which imaging modality—CT, MRI, or PET (see below)—should be performed in patients who require or would likely benefit from additional surveillance beyond the “first-line” tests of serum thyroglobulin and cervical ultrasound. The decision is made based on a number of factors, including but not limited to suspected or most likely site of residual/recurrent disease, method by which extrathyroidal disease was first detected (if applicable), anticipated need for diagnostic or therapeutic iodine in the near future, preexisting renal disease, relative costs of each imaging technique, and access to these imaging modalities.
FDG-PET Scanning
FDG-PET imaging is a functional nuclear medicine test in which PET scanning is used to localize the uptake of 18F-fluorodeoxyglucose (FDG) following its intravenous injection. Metabolically active tissue utilizes glucose to a greater degree than inactive tissue, a principle that underlies the rapid expansion and now routine use of FDG-PET in staging and monitoring throughout oncology [7]. Unlike many other malignancies, however, most differentiated thyroid cancer is slow growing and lacks high levels of metabolic activity. Thus, FDG-PET is not a first-line staging or surveillance tool for most patients with DTC. In fact, the sensitivity of FDG-PET even in patients with a modestly elevated thyroglobulin is low, ranging from <10% to at most 30% in patients with a stimulated thyroglobulin of <10 ng/mL [129, 130].
However, FDG-PET is useful for detection of disease in high-risk DTC patients with serum thyroglobulin >10 ng/dL and negative radioiodine scans. The first report of localization of thyroid cancer by FDG-PET, published in 1987 [131], and several subsequent larger series of patients with DTC [132–134], all showed that FDG-concentrating lesions were more aggressive in their growth pattern than those that concentrated only radioiodine and that an inverse relationship between FDG avidity and radioiodine avidity existed for most of the detected lesions. This relationship may be explained by loss of the ability to transport and/or organify iodide but a gain in glucose transporter expression in aggressive thyroid cancers, leading to reduced visibility on 131I scanning while simultaneously increasing visibility on FDG-PET [7]. Dietlein et al. found that FDG-PET was 82% sensitive for localizing disease in patients with significantly elevated serum thyroglobulin concentrations and negative radioiodine scans [135]. Multiple subsequent studies confirmed these findings, and a study by Wang et al. demonstrated an approximate 92% positive predictive value of FDG-PET in thyroglobulin-positive, radioiodine scan-negative patients [136]. Sensitivity is higher with greater degrees of dedifferentiation and larger tumor burden. Sensitivity has also been reported to be particularly high (92%) in Hurthle cell cancer, perhaps related to the extremely high concentrations of mitochondria in Hurthle cells [137–139]. Most thyroid cancer lesions missed by FDG-PET are due to small-volume cervical lymph nodes, which may be detected by high-resolution neck ultrasound.
The addition of TSH stimulation, either by THW or rhTSH, modestly increases the sensitivity of FDG-PET. Biologically, this effect can be attributed to the ability of TSH to increase glucose uptake and metabolism in thyroid cells [23]. In published studies, TSH stimulation has led to the identification of additional FDG-avid lesions compared to unstimulated conditions, but generally has not made a difference in finding versus not finding any FDG-avid lesion, and overall has led to a change in management in only 6–9% of cases [129]. An additional benefit of TSH stimulation, beyond the detection of additional foci of FDG-avid DTC, is a possible improvement in the ability to distinguish FDG-PET-positive thyroid cancer tissue from lymph nodes or other FDG-avid tissues, i.e., increasing the test’s specificity [140, 141]. On the basis of available data, TSH stimulation should be considered on a case-by-case basis, but is not essential for the use of FDG-PET as a surveillance tool in thyroid cancer.
Beyond its role in surveillance, FDG-PET is useful in several aspects of thyroid cancer management that are addressed in other chapters. These include initial staging of high-risk thyroid cancer and prognostication, planning therapy for known disease, targeting cancers at highest risk of progression, and following treatment response.
Drawbacks to use of FDG-PET as a surveillance tool include its expense, as well as its relatively poor specificity compared to more thyroid tumor-specific modalities such as radioiodine scan. The specificity can be improved by combining FDG-PET with CT (FDG-PET/CT), which may, for example, allow reactive lymph nodes to be distinguished from cancerous ones, but there is still a frequency of up to 39% for false-positive lesions with FDG-PET in published series [121, 140]. For this reason, it is important to confirm a positive finding on FDG-PET with fine-needle aspiration (using cytology and Tg-FNA) before proceeding to treatment.
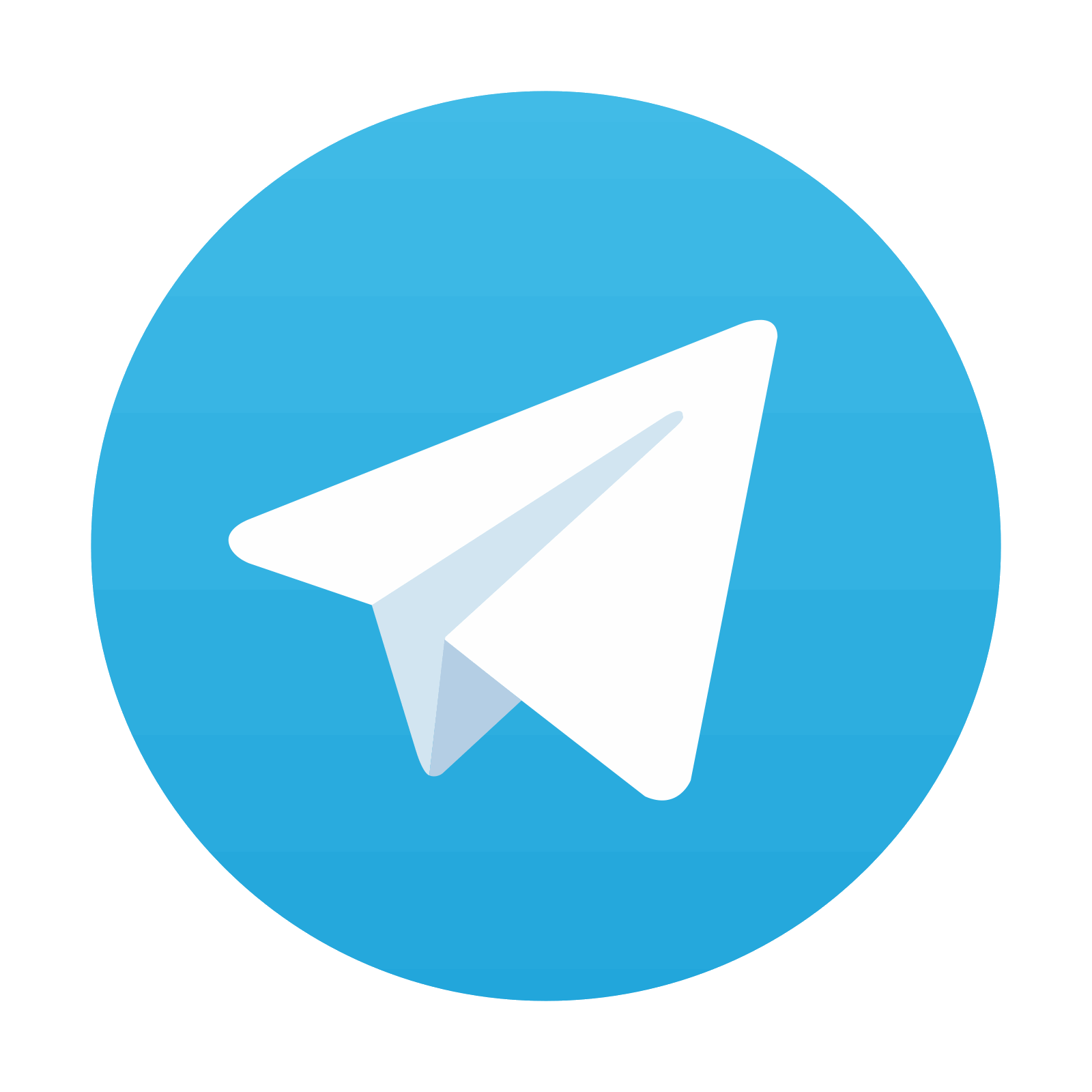
Stay updated, free articles. Join our Telegram channel
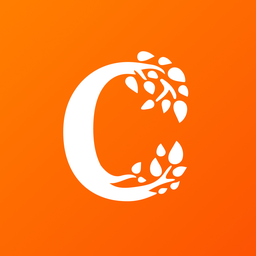
Full access? Get Clinical Tree
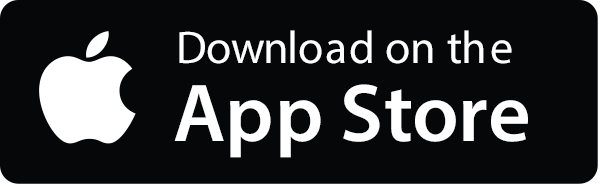
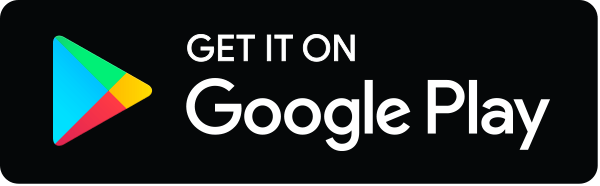