Predictor
Score
Age < 2 years
3
Presence of transependymal edema
1
Moderate/severe hydrocephalus
2
Cerebral metastases
3
Preoperative estimated tumor diagnosis
Medulloblastoma
1
Ependymoma
1
Dorsally exophytic brainstem glioma
1
Total possible
10
Prompt recognition of hydrocephalus is critical. In a nonverbal patient, the first indication is often that the parents notice lethargy, irritability, nausea, vomiting, or a seizure (Shiminski-Maher and Disabato 1994). In a patient who is able to communicate, headaches are the cardinal symptom. The headaches are worse in a recumbent position at night or on first rising in the morning and improved with sitting or standing throughout the day. Bradycardia probably results from pressure on the dorsal motor nucleus of the vagus nerve in the medulla and on the nucleus ambiguous, which contains preganglionic parasympathetic neurons whose axons travel with the vagus nerve (Siegel et al. 2015). Papilledema is a key physical finding and almost always indicates that intracranial pressure (ICP) is elevated. However, its absence does not exclude elevated ICP as papilledema can take several days to develop (Hayreh and Hayreh 1977; Steffen et al. 1996). In cases where the physical finding of papilledema is in doubt and the rest of the workup is equivocal, evaluation by an ophthalmologist may be necessary. Other physical findings of subacute/chronic hydrocephalus include distended scalp veins, upward gaze palsy from pressure on the midbrain, a bulging fontanelle, and a head circumference that crosses percentiles. In infants with an open fontanelle, the initial symptoms may be more subtle since the open fontanelle initially provides compliance against changes in ICP.
Cerebral imaging often provides the first indication of hydrocephalus in a cancer patient. On CT scans, ventriculomegaly is the obvious sign and, in acute cases, there is a distinct hypodense-blurred margin to the ventricles. The third ventricle is the most compliant; often, the first radiographic sign of increased ventricular pressure is a distended or “rounded” third ventricle. Cortical sulcal effacement may occur with large ICP elevations. T2 MRI sometimes shows hyperintense transependymal CSF flow, which is especially prominent around the ventricular horns. The corpus callosum may be thinned and stretched upward. Quantitative measures include an Evan’s ratio (ratio of the bifrontal horn diameter to the intracranial diameter) greater than 1/3 and a temporal horn width greater than 3 mm (Osborn 2013). In practice, the Evan’s ratio is more useful for trending ventricle size rather than diagnosing hydrocephalus since the diagnosis is usually apparent from other radiographic features.
Procedures for treating hydrocephalus are among the most established in the field of neurosurgery. They include the external ventricular drain (EVD or “ventriculostomy”), ETV, and shunts. The most rapid method for treating obstructive hydrocephalus is an EVD. This procedure can be done in the operating room or (in emergency circumstances) at the bedside with local anesthesia. It utilizes a frontal approach to target drain placement near the foramen of Monroe. The distal tip of the ventricular drain is tunneled under and out through the skin and connected to a reservoir to collect CSF through the siphon effect of gravity. CSF is drained either by pressure (0–20 cm above the ear of the patient) or by volume (a fixed volume of 10–15 cc per hour). Under or over drainage of CSF should be avoided. The EVD is an ideal choice in several scenarios: acute hydrocephalus to temporize the patient pending operating room availability or pending operative planning for complex tumors, when hydrocephalus is known to be due to a cause that will soon be reversed (such as a fourth ventricle obstructive tumor), or when diagnostic ICP readings are necessary if the diagnosis of hydrocephalus is unclear but strongly suspected (lumbar puncture opening pressure is not a reliable indicator of ICP in noncommunicating hydrocephalus and may precipitate life-threatening herniation).
Long-term treatments for hydrocephalus are the ETV and ventricular shunts. ETV involves endoscopic navigation via a cranial burr hole to the floor of the third ventricle and fenestration of the third ventricle through the tuber cinerium. This provides an alternate route of egress, as CSF can flow directly from the third ventricle to the pre-pontine cistern after this procedure is performed. The complications of an ETV are primarily periprocedural. Visual obscuration of the endoscopic camera can be caused by even a slight amount of intraventricular blood. Coagulating the bleeding vessel is difficult through a single channel endoscope. Damage to the fornices may occur with devastating clinical outcomes, including the loss of short- and long-term memory. Hypothalamic damage can manifest as diabetes insipidus, amenorrhea, change in appetite, growth hormone disturbance, hypothyroidism, loss of thirst, electrolyte abnormalities, or other components of hypothalamic-pituitary axis disruption (Bouras and Sgouros 2011). Damage to the basilar artery, which has a variable location in relation to the floor of the third ventricle and is sometimes difficult to visualize at the time of puncture, can be fatal. Postoperative complications include CSF leak (3.1%), meningitis (2.3%), hemorrhage (1.7%), subdural CSF collection (1.7%), and seizure (1%) (Sacko et al. 2010). Furthermore, an ETV may obstruct at any time, but the majority of failures occur within the first 6 months after surgery; in patients with aqueductal stenosis or tumors, there may be a second “peak” of failures 3 years after surgery (Lam et al. 2014).
Ventricular shunts are among the most well-established procedures in the field of neurosurgery. They usually utilize either a frontal or occipital entry point to target the lateral ventricle. A peritoneal termination is usually favored (ventriculoperitoneal shunt) with enough catheter length coiled in the abdominal cavity to account for the child’s growth, but other sites can be used, including the heart (ventriculoatrial shunt), lung (ventriculopleural shunt), or gallbladder (least commonly used). Non-peritoneal sites are usually used after several prior peritoneal insertions have been performed, after which peritoneal adhesions will develop. CSF diversion from the lumbar spine to the peritoneum (lumboperitoneal shunt) is also an option in patients with communicating hydrocephalus. Shunts carry a high risk of failure. In a study of new shunts placed in pediatric patients, there was a 40% risk of failure in 1 year and a 50% risk of failure in 2 years. Overall, there is a 5% risk of failure per year. Obstruction (31%) and infection (8%) are the most common causes of failure, but after 6 months, the infection risk is miniscule (Drake et al. 1998). Compared to endoscopic techniques, shunts have a low upfront cost, but the cost increases over time. Retrospective data showed that 42% of shunt procedures are shunt revisions. Of the $100 million spent on caring for shunt patients each year, about half is spent on revisions (Bondurant and Jimenez 1995). Shunts also carry a significant risk of seeding tumor at the distal site; a recent meta-analysis showed that 27% of cases of primary brain tumors in children that metastasized were related to shunt placement, with germ cell tumors being the most common shunt-related metastasis (Rickert 2003). Due to the major complications associated with shunt placement in patients with brain tumors, the decision to commit a patient to a shunt requires careful consideration. If a shunt is placed, programmable valves susceptible to the effects of the strong magnetic field in an MRI scanner should be avoided, as patients with brain tumors usually require serial MRIs into the foreseeable future. If a programmable shunt valve is used, patients need a skull X-ray (orthogonal to the shunt valve such that its setting can be determined) before and after each MRI to ensure that the valve settings was not inadvertently changed by the strong magnetic waves.
When endoscopic capabilities are available to a neurosurgeon, the decision of whether to perform an ETV or shunt can be aided by the ETV success score (Table 4.2) (Kulkarni et al. 2009). The ETV success score assigns a higher likelihood of ETV success by 6 months for older children, children with brain tumors (especially tumors causing aqueductal stenosis), and patients with no previous shunt. Since an ETV is often technically difficult in newborns and infants, a practical derivation of the ETV success score is that for a child 6 months or older with tumor-related hydrocephalus, an ETV would be expected to have at least 50% success rate by 6 months. Because placing a shunt commits a patient to a high near-term risk of infection and an unremitting risk of obstruction, some practitioners tend to give patients a trial of an ETV before resorting to a shunt. The role of ETV in the management of hydrocephalus is actively being studied. As technological advancements arrive (including flexible endoscopes capable of entering the temporal horns, which will allow near full coagulation of the CSF-producing choroid plexus), ETV will gain a greater role in treating hydrocephalus. A recent study showed that when ETV is combined with partial choroid plexus cauterization, the 6-month success rate is improved from 45% expected from the ETV success score to 59% (Stone and Warf 2014).
Table 4.2
The Endoscopic Third Ventriculostomy (ETV) Success Score. Age score + etiology score + previous shunt score = estimated percentage probability of ETV success at 6 months after surgery
Score | Age | Etiology | Previous Shunt |
---|---|---|---|
0 | <1 month | Postinfectious | Yes |
10 | 1 month to <6 months | No | |
20 | Myelomeningocele, intraventricular hemorrhage, non-tectal brain tumor | ||
30 | 6 months to <1 year | Aqueductal stenosis, tectal tumor, other etiology | |
40 | 1 year to <10 years | ||
50 | ≥10 years |
Rapid recognition of ETV failure or shunt failure is critical, and if there is any concern for failure, a neurosurgeon must be consulted. The radiographic finding of newly enlarged ventricles in a patient with a shunt or ETV indicates failure; no other workup is necessary. However, many times comparison CT scans are not available or the CT scan is equivocal for ventricular dilatation. Also, patients with shunt failure do not always have dilated ventricles (Sellin et al. 2014). In such cases, one must rely on the clinical history and physical exam to diagnose the failure, or a shunt tap of the reservoir. If an ETV was performed, a CINE MRI scan of the brain can be performed to evaluate for flow through the fenestration in the floor of the third ventricle.
In the uncommon case that a patient presents with acute hydrocephalus due to distal shunt failure (usually symptoms of distal failure are more indolent), a shunt tap to remove CSF may be lifesaving. When distal failure of a ventriculoperitoneal shunt is suspected, an abdominal CT is often performed to check for a pseudocyst, infection, or very uncommonly tumor seeding at distal end of the shunt. In cases of symptomatic shunt obstruction, the only definitive treatment is emergent shunt revision or temporization with an EVD.
4.4 Posterior Fossa Syndrome
In 1985, Rekate reported several cases of mutism in children after posterior fossa surgery (Rekate et al. 1985). Since then, there have been many reports of mutism following surgery for cerebellar tumors. The posterior fossa syndrome (PFS) includes various specific linguistic, cognitive, and behavioral symptoms that occur after surgery for lesions of the cerebellum. Symptoms typically develop a few days after an otherwise normal postoperative course after resection of a posterior fossa tumor in a child (Wells et al. 2008). (PFS has been reported in adults, but it is uncommon (Marien et al. 2013)) The hallmark symptom of PFS is mutism (“cerebellar mutism”), which is often accompanied by frontal-like behavioral symptoms such as abulia, apathy, or lack of concern (Levisohn et al. 2000). After remission of the mutism, patients continue to be dysarthric; speech is present but impaired by motor difficulty, and this rarely normalizes (Wells et al. 2008). Hence, PFS is sometimes called “mutism and subsequent dysarthria syndrome.” Importantly, there are no long tract signs and no supranuclear or cranial nerve palsies (Wells et al. 2008). The presenting symptoms are usually transient and resolve with time, but long-term adverse sequela may include decline of general intelligence and scholarly underachievement (Steinlin et al. 2003; De Smet et al. 2009). PFS was initially reported as “rare,” but a recent prospective series of patients undergoing medulloblastoma resection reported an incidence of 24% (Robertson et al. 2006).
The pathophysiology behind PFS has not yet been determined. Two main hypotheses, which are not mutually exclusive, have been advanced to explain the symptoms of PFS: interruption of the dentatothalamocortical pathway due to bilateral damage to the dentate nuclei and cerebellar swelling.
4.4.1 Interruption of the Dentatothalamocortical Pathway as a Possible Cause of PFS
The dentatothalamocortical pathway is the primary outflow tract from the cerebellum. It originates at the dentate nucleus, sends projections through the ipsilateral superior cerebellar peduncle, decussates in the midbrain tegmentum, and synapses in the contralateral ventrolateral thalamus. There, synapses occur with second order neurons that project to the supplementary motor area (Kandel 2013). Myelination is incomplete in children, and this may lead to greater susceptibility to disturbances of this pathway in younger patients (Ildan et al. 2002). Crutchfield observed that lesions along this pathway lead to mutism (Crutchfield et al. 1994): Mutism has been reported after stereotactic lesioning of both dentate nuclei for treatment of a dyskinetic syndrome (Fraioli and Guidetti 1975). Mutism has been reported after bilateral thalamotomy for Parkinson’s disease (Siegfried et al. 1970). Finally, lesions of the supplemental motor area can also cause mutism (Crutchfield et al. 1994). MRI studies of patients with PFS often show evidence of proximal injury along this pathway (Morris et al. 2009). In fact, a recent anatomical study demonstrated in cadavers the high susceptibility of the dentate nuclei to damage during various posterior fossa surgical approaches (Akakin et al. 2014). Sagging of the cerebellum into a postsurgical resection cavity may cause distortions of these tracts as well, exacerbating injury. Crutchfield observed that when lesions occur in this pathway, symptomatic recovery (when it occurs) is usually quite sudden—from mutism to the immediate return of words and sentences (Crutchfield et al. 1994). From this clinical observation, the authors hypothesized that lesions along this pathway cause an inhibition of cortical language centers, and recovery reverses the inhibition. It is possible that the dentatothalamocortical pathway contributes a permissive input to cortical language circuitry that, when restored, allows rapid recuperation of linguistic ability.
4.4.2 Cerebellar Swelling as a Possible Cause of PFS
Ferrante proposed that postoperative vasospasm of cerebellar arteries could cause ischemia and subsequent cerebellar swelling and edema (Ferrante et al. 1990). To date, there have been no clinical studies of cerebral angiography after posterior fossa surgery to evaluate for vasospasm. SPECT studies have shown hypoperfusion of the left cerebellar hemisphere in a patient with PFS, and the abnormality resolved together with the mutism (Clerico et al. 2002; Ersahin et al. 1996). A recent series also showed that 92% of patients with PFS had postoperative cerebellar edema, and the edema often affected the middle and superior cerebellar peduncle (Wells et al. 2010). The same study showed that patients who developed PFS often had tumors that invaded into the brainstem. It is conceivable that the cerebellar swelling may result from retraction and other manipulations during surgery and not necessarily from vasospasm.
Splitting of the vermis, especially the inferior part, has been suggested to cause PFS (Dailey et al. 1995). In fact, the telovelar approach for resecting posterior fossa tumors was developed specifically to avoid splitting the vermis (Mussi and Rhoton 2000). However, this association between splitting the vermis and PFS is questioned by some modern authors (Pitsika and Tsitouras 2013). Robertson showed no correlation between vermis tumor location and the development of PFS (Robertson et al. 2006). They noted that the majority of patients with posterior fossa tumors underwent radical resection through the vermis and did not develop mutism afterward. Hydrocephalus had, at one time, been proposed as the cause of PFS (Ferrante et al. 1990). However, later studies showed no association between hydrocephalus and PFS (Crutchfield et al. 1994; Catsman-Berrevoets and Aarsen 2010).
4.5 Mass Effect/Intracranial Pressure Management
Several temporizing measures can be employed to treat tumor-related mass effect and the associated increase in ICP, pending definitive treatment. The head of the bed should be elevated to 30–45°. This reduces ICP by enhancing venous outflow but, at the same time, slightly diminishes MAP and cerebral perfusion pressure. The head should face straightforward (not turned to one side)—this also enhances venous drainage. The first-line pharmacological agent for the non-emergent treatment of tumor-associated mass effect is steroids, usually dexamethasone (discussed later in this chapter). Hyperventilation lowers ICP by reducing the partial pressure of CO2, which, by various physiologic mechanisms, reduces cerebral blood volume. Hyperventilation can be used for brief periods of time with a pCO2 goal of 30–35 but should not be used for long-term treatment of elevated ICP, as it places patients at risk for cerebral ischemia. The mechanism by which mannitol lowers ICP is controversial, but it may involve improved rheology (plasma expansion reduces the hematocrit, which increases cerebral blood outflow) (Burke et al. 1981). Diuresis and osmotic effects are more delayed and take 15–30 min for onset. In urgent situations, 1 g/kg of mannitol can be given intravenously over 30 min. Hypertonic saline also has an osmotic effect, and 3% saline is often given therapeutically through a central line with serum sodium checks performed every 6 h to maintain sodium levels of 145–155.
4.6 Steroids
Glucocorticoids are a well-established treatment for tumor-associated edema. They are frequently used in the following scenarios: (1) to temporize a patient who has a surgically resectable brain tumor while the definitive resection is being planned; (2) to reduce swelling in the perioperative period (after the operation, the dose is tapered down); (3) in combination with certain chemotherapy regimens to reduce edema during administration of the therapeutic agent; (4) to minimize swelling during radiation therapy; and (5) to palliate swelling in the setting of an inoperable brain tumor. Clinically, a response is usually seen within a few hours (because the pharmacologic action of steroids modifies DNA transcription (Ratman et al. 2013)). Thus, steroids are not the first-line agent for a tumor-associated ICP crisis (Alberti et al. 1978). Dexamethasone is the most commonly used glucocorticoid for treating tumor-associated cerebral edema, as its low level of mineralocorticoid activity reduces the potential for fluid retention (Galicich et al. 1961). Dexamethasone may also have a lower risk of infection and cognitive impairment compared to other steroids (Batchelor and DeAngelis 1996). Steroids are effective in treating vasogenic edema, which occurs due to disruption of the blood–brain barrier (Bebawy 2012). In contradistinction, they are not effective in treating cytotoxic edema, which occurs due to cellular swelling (such as the edema that occurs after a stroke).
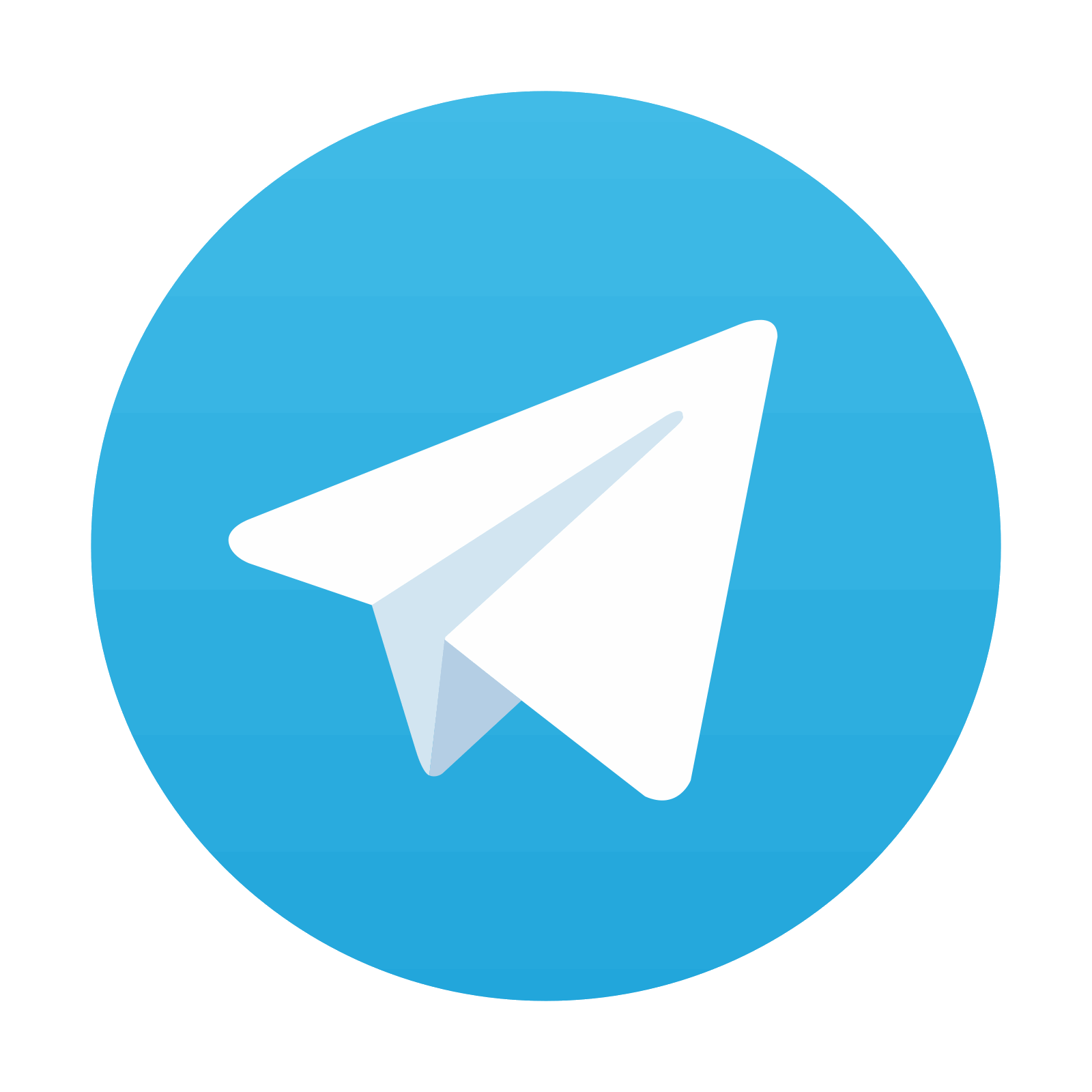
Stay updated, free articles. Join our Telegram channel
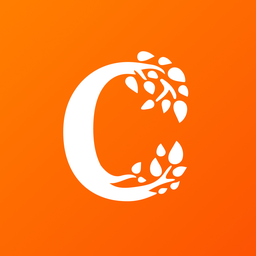
Full access? Get Clinical Tree
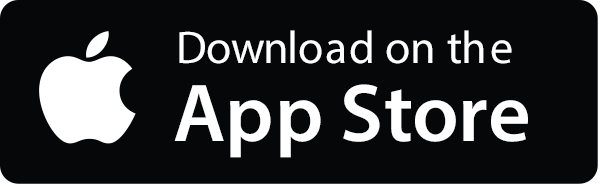
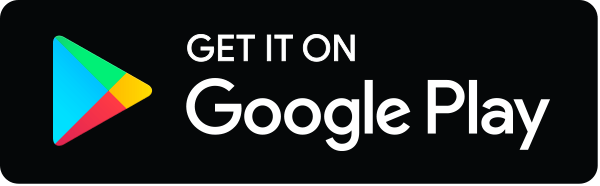