2 Structure and Function of the Nervous System
The Somatic Nervous System
N. E. Cameron
Overview
The somatic nervous system is subdivided into motor and sensory components. The motor division is concerned with control of skeletal muscle contraction, and hence of voluntary movement and of posture and reflexes. The somatosensory division is a collection of receptors, tracts, and nuclei that convey the sensations of light touch, vibration, temperature, and pain (nociception) to the consciousness. It also conveys information about movements and position of the body (proprioception and kinesthesia). Somatosensory receptors are found in the skin, muscles, joints, and viscera. In addition to providing sensation, the somatosensory division has a critical role in motor control, through feedback about muscle length and tension, joint position, velocity of muscle and limb movement, and contact with external surfaces. The basic structure and function of the somatic nervous system has been described in numerous physiology, neurology, and neuroscience texts: the reader is referred to two recent books for further information [1,2]. Diabetes affects both the peripheral and the central nervous system (for discussion of the latter see Chapter 5, page 205–208), although most clinical and scientific interest has focused on the periphery because of the devastating effect on nerve fiber integrity. This section provides an overview of the substrate of the somatic nervous system, with a greater emphasis on peripheral than on central structures.
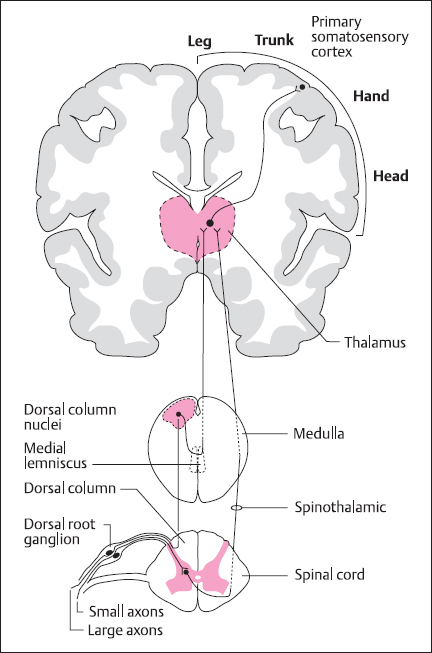
Fig. 2.1 General organization of the somatosensory system, showing the dorsal column-lemniscal system which mediates touch sensation and proprioception, and the spinothalamic system, which deals with temperature and fast pain information
Central Nervous System Pathways
Somatosensory System
There are two major somatosensory pathways running from the spinal cord to the primary sensory area (area 3 or S1) on the postcentral gyrus of the cerebral cortex (Fig. 2.1). Information about touch and proprioception is carried in the dorsal column medial lemniscus system, whereas temperature and pain information traverses the spinothalamic pathway [3].
In the touch system, fibers from first-order neurons with cell bodies in the dorsal root ganglia traverse the dorsal columns of the spinal cord (Fig. 2.1) to the dorsal column nuclei within the caudal medulla. Of these, the cuneate nucleus receives input from the upper limbs and body whereas the gracile nucleus deals with fibers from the lower limbs. Second-order neurons then project across the midline as the “sensory decussation” and ascend via the medial lemniscus to the ventral posterolateral nucleus of the thalamus. Touch information from the head follows a parallel route, with the first major relay in the principal trigeminal nucleus of the pons, with mid-pontine decussation to reach the ventral posteromedial nucleus of the thalamus via the ventral trigeminal tract. The inputs from the various regions of the body are segregated and aligned such that the pathway is somatotopically organized. This is reflected by the cortical projection (Fig. 2.1), where there is an orderly mapping of the contralateral side of the body on the cortical surface.
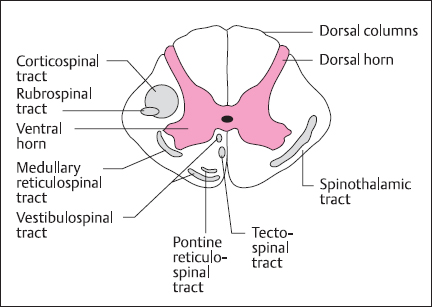
Fig. 2.2 Schematic cross-section of spinal cord showing the gray matter and white matter containing the major sensory (right) and motor (left) nerve tracts
Information about temperature and pain (particularly the fast component) is transmitted by the spinothalamic tract. Here, the first synaptic relay is in the gray matter of the spinal cord; projection fibers then decussate in the ventral white commissure to form the contralateral spinothalamic (or anterolateral) tract (Fig. 2.2).These reach the ventral posterolateral nucleus of the thalamus, and some fibers also project to the small thalamic intralaminar nuclei. Parallel pathways transmit information from the face, via the spinal trigeminal nucleus, to the ventral posteromedial thalamus. The pathway depicted in Figure 2.1 is also known as the neospinothalamic pathway, and there are other, phylogenetically older pathways transmitting pain information (particularly the slow, poorly localized, long-lasting component). These are the paleospinothalamic and spinoreticulothalamic pathways, which are polysynaptic and ascend through the reticular formation to nonspecific nuclei in the medial thalamus and intralaminar nuclei. They project to widespread regions of cerebral cortex, rather than being associated with the somatotopic organization of the primary sensory cortex: this may contribute to the poor ability to localize slow pain.
Motor System
There are a number of important fiber tracts (Fig. 2.2) descending from the brain to control the activity of ventral horn motoneurons supplying the skeletal muscles [2]. These can be divided into lateral and ventromedial groups. The lateral pathway comprises corticospinal and rubrospinal tracts and is primarily involved in voluntary movement, particularly of the distal muscles, under direct cortical control. The ventromedial pathways originate in the brainstem, forming reticulospinal, tectospinal and vestibulospinal tracts, which are involved in the control of posture and locomotion.
The most important component of the lateral pathway is the corticospinal tract, which originates primarily from areas 4 (primary motor cortex or MI) and 6 (supplementary motor area) of the frontal lobe, on the precentral gyrus, located across the central sulcus from the primary somatosensory cortex. The motor cortex is somatotopically organized, and axons pass through the int9rnal capsule and course through the midbrain and pons to form a pyramid-shaped tract running down the ventral surface of the medulla. At the junction with the spinal cord, the pyramidal tract decussates. Thus, as in the somatosensory system, the right motor cortex processes information for the left side of the body and vice versa. The axons from the motor cortex then group to form the lateral corticospinal tract and terminate in the dorsolateral and intermediate gray matter region of the ventral horns, where the motoneurons and interneurons that control the distal muscles are located.
The rubrospinal tract is a much smaller component of the lateral pathway and originates in the red (Latin ruber) nucleus of the midbrain. Axons decussate in the pons and join the corticospinal tract in the lateral columns of the spinal cord. The red nucleus itself receives its major input from the motor areas of cerebral cortex, which also give rise to the corticospinal tract. While the rubrospinal tract is important in many mammalian species, in man much of its function has been taken over by the corticospinal pathway.
The ventromedial pathways may be divided functionally into two groups: the tectospinal and vestibulospinal tracts control the posture of head and neck, whereas the pontine and medullary reticulospinal tracts control the posture of the trunk and limb antigravity muscles. The vestibulospinal tract originates in the medullary vestibular nuclei, which are involved with processing sensory activity from the vestibular apparatus of the inner ear. In combination with proprioceptive information about body and neck position, this pathway is importantly involved in maintaining head-body alignment to ensure that the eyes and our image of the world remain stable [4]. The tectospinal tract originates in the superior colliculus of the midbrain. This structure receives direct input from the retina as well as the visual cortex and auditory systems. It is involved with the coordination of head and eye movements and orienting responses towards stimuli [5].
The pontine reticulospinal tract acts to facilitate the antigravity reflexes of the spinal cord to aid the maintenance of a standing posture by promoting extensor activity in the lower limbs and flexor activity in the upper limbs. The medullary reticulospinal tract has an opposite action, to inhibit reflex domination of anti-gravity muscles, thus allowing greater control by lateral pathways. The balance of activity in these reticulospinal tracts is controlled by descending signals from motor cortex [2].
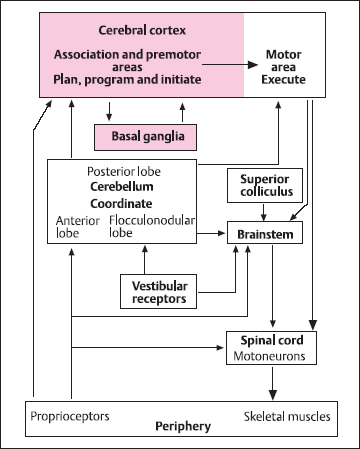
Fig. 2.3 Schematic of motor control showing the major cortical and subcortical regions of the central nervous system. The association and premotor areas of cerebral cortex, along with the basal ganglia, are responsible for planning and initiating voluntary movements. The motor cortex, and its direct connection with the α-motoneurons in the spinal cord, is responsible for sending the appropriate instructions for execution of the movement by the skeletal muscles. The cerebellum provides information about coordination, sequencing, and timing of complex movements. Brainstem mechanisms, along with the vestibular apparatus, superior colliculus, and older areas of the cerebellum, have a major role in the control of posture and gait
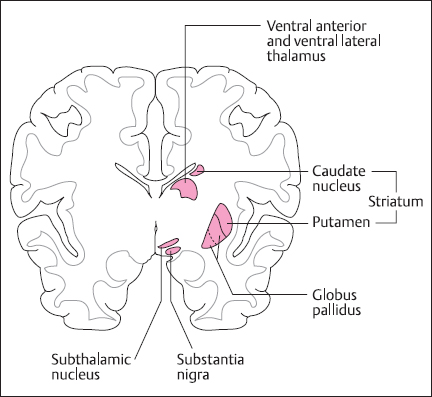
Fig. 2.4 Cross-section of the brain showing the location of the major nuclei that contribute to basal ganglia function upper limbs. The medullary reticulospinal tract has an opposite action, to inhibit reflex domination of antigravity muscles, thus allowing greater control by lateral pathways. The balance of activity in these reticulospinal tracts is controlled by descending signals from motor cortex [2].
While cortical areas 4 and 6 comprise the motor cortex, in terms of the control of voluntary movement many other areas of the cerebral cortex are involved as well as important subcortical structures such as the basal ganglia and cerebellum. The overall organization of the motor control system is outlined in Figure 2.3. Thus, the processes of planning, programming, and initiating goal-directed movement involve association and premotor cortical areas, including the anterior frontal lobes and regions of the posterior parietal cortex such as area 5, which gets a direct input from somatosensory cortex, and area 7, which receives connections from higher-order visual cortical areas.
The major subcortical input to area 6 of motor cortex arises from the ventral lateral nucleus of the thalamus, which in turn comes from the basal ganglia (Fig. 2.4). These comprise the caudate nucleus and the putamen (collectively termed the striatum), the globus pallidus, the subthalamus, and the midbrain substantia nigra. These areas form a complex circuit that funnels or focuses activity from widespread areas of association cortex on to area 6, perhaps supplying basic motor programs for the desired action. This cortex → striatum → globus pallidus → thalamus → motor cortex loop is an integral part of the movement initiation process, and the other structures form side loops that modulate this pathway [6].Diseases of basal ganglia result in problems with voluntary movement initiation, involving hypokinesia, as in Parkinson’s disease, or hyperkinesias, as in Huntington’s disease. In addition to their role in initiation, basal ganglia also modulate posture and muscle tone, abnormalities of which are found in basal ganglia disease. The basal ganglia also have a role in nonmotor behaviors, including cognition and mood [7].
The cerebellum is involved with coordination of the sequence of muscle contractions during a movement. In man, there are three important functional subdivisions of the cerebellum. The anterior lobe (paleocerebellum, spinal cerebellum) and its associated deep cerebellar nuclei (fastigial, interposed, and lateral vestibular nuclei) is concerned primarily with processing of information from muscle, joint, and cutaneous mechanoreceptors. It also receives input pertaining to activity in the motor cortex via pontine nuclei and collaterals of corticospinal fibers. The anterior lobe output provides information to modulate the brainstem nuclei from which the reticulospinal tracts originate, and there is a projection to the red nucleus. These circuits are involved in the control of posture and gait.
The flocculonodular lobe (archicerebellum, vestibular cerebellum) and fastigial nucleus are involved with the coordination of the paraxial muscles associated with balance and equilibrium. The major input comes from the vestibular apparatus, and output goes to the vestibular nuclei and then to the vestibulospinal tracts. There is also an important vestibulo-ocular projection to the external ocular muscles.
The posterior lobe (neocerebellum, cerebral cerebellum) and dentate nucleus have massive reciprocal connections with cerebral cortex, including motor, premotor, sensory, and posterior parietal areas. This cerebellar subdivision is involved with coordination of voluntary movement sequences, particularly ballistic movements that are normally too fast to be under feed-back proprioceptive control. It is responsible for smooth and accurate execution of movements, and shows evidence of the synaptic plasticity necessary for the learning and refinement of complex motor skills [8].
Peripheral Nerve, Receptors, and Spinal Cord
Peripheral Nerve Fiber Types
Peripheral nerve consists of bundles of nerve fibers, generally mixed sensory and motor. Fiber types have been classified in two ways [2]. The first depends on axon diameter, with categorization into groups A, B, and C. The largest axons, which are myelinated, belong to group A. The smallest fibers, which are unmyelinated, belong to group C. The B group contains myelinated axons from autonomic preganglionic neurons, although this classification is rarely used today. The A group is further classified into the subgroups α, β, δ, and γ.
There is also a second classification system for some of the sensory axons, based primarily on conduction velocity, but also on origin and function. This categorization has numerical classes I–IV in descending order of conduction velocity. Because conduction velocity is directly related to axon diameter for myelinated fibers, the two classification systems can be related as shown in Table 2.1. Both terminologies are in common usage, although they were developed independently and do not overlap exactly in terms of fiber categories.
Somatosensory Receptors and Sensation
Somatosensory receptors can be divided into three groups: mechanoreceptors, thermoreceptors, and nociceptors (Table 2.2). Mechanoreceptors respond to deformation of their nerve endings, which contain specialized mechanosensitive ion channels whose gating depends on stretching or changes in tension of the surrounding membrane. The nerve endings of mechanoreceptors are usually associated with specialized nonneural structures that govern their detailed response characteristics, so determining the adequate stimulus. Mechanoreceptors mediate the sensations of light touch, pressure, vibration and flutter, and limb position and movement (kinesthesia). Examples of mechanoreceptors in hairy and hairless (glabrous) skin are shown in Figure 2.5.
Cutaneous mechanoreceptors [9] have punctate receptive fields whose size is determined by the area of nerve terminal branching and associated nonneural tissue. The information and sensation gleaned from these receptors is governed by their degree of adaptation to a constant stimulus. They may be classified into slowly adapting, moderately rapidly adapting, and very rapidly adapting categories. Slowly adapting receptors respond with an increased frequency of action potentials for the duration of a stimulus. Thus, they are able to accurately signal skin indentation and pressure. Merkel’s disk receptors and Ruffini’s endings fall into this category. Moderately rapidly adapting receptors respond with a burst of action potentials at stimulus onset and comprise the Meissner’s corpuscle of glabrous skin and the hair receptor. They are best at signaling the velocity of movement of a stimulus, and are most sensitive to low-frequency (<50 Hz) repetitive stimulation. In this frequency range, a sinusoidal mechanical stimulus will give rise to the sensation of “flutter” where individual waves of the vibration are felt. This contrasts with higher frequencies, which are felt as a true unitary vibration, and this information is transmitted by the most rapidly adapting receptor type, the pacinian corpuscle.
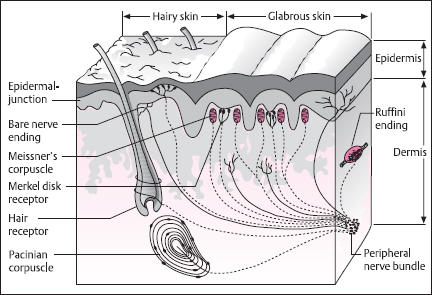
Fig. 2.5 Schematic of hairy and glabrous (hairless) skin, showing the location of various mechanoreceptors. The receptors in glabrous skin are Meissner’s corpuscles and Merkel’s disks, located in the dermal papillae, and bare nerve endings. In hairy skin, there are hair receptors around the hair shafts. Merkel’s disks, and bare nerve endings. Beneath both types of skin, in the subcutaneous region pacinian corpuscles and Ruffini’s endings are found. (From [1], with permission)
The different receptor types work together along with hand movements and skin patterns such as fingerprints for shape and texture discrimination [10]. Thus, while the discharge of slowly adapting Merkel’s disks is best at encoding the spatial characteristics of a stimulus, the more rapidly adapting receptors provide texture information from vibrations set up by the mechanical interaction between surface and fingerprints as the finger tip is moved across a surface.
The sensitivity of the skin to mechanical stimulation varies widely over the body [1], as can be seen from the results of two-point spatial discrimination tests (Fig. 2.6). Thus, highest sensitivity is noted for the fingers, lips, nose, and toes, whereas the trunk and upper limbs are relatively insensitive. This is a direct reflection of peripheral innervation density, the number of receptors per unit area of skin, and the average size of individual neural receptive fields, which are correspondingly larger in regions of low sensitivity.
The muscle and skeletal mechanoreceptors comprise muscle spindles, joint receptors, and Golgi tendon organs [11,12]. They are known as proprioceptors because they convey information on the position and movement of the limbs. The major receptor is the muscle spindle (Fig. 2.7), formed from specialized skeletal muscle fibers, the intrafusal fibers. Sensory endings contact the midsection of these fibers in an area devoid of contractile machinery. These afferents are stimulated by stretch and signal muscle length and velocity of lengthening. There is also an efferent supply from specialized γ-motoneurons that innervate the contractile ends of the intrafusal fibers. When activated, this causes the ends to contract and in so doing stretches the noncontractile element, including the afferent endings. The function of this efferent system is to regulate the sensitivity of the afferent fibers during active muscle contractions. In the execution of voluntary movements, when activity is supplied to the contractile (extrafusal) fibers of skeletal muscle via the α-motoneurons, there is also modulatory impulse traffic in the γ system – the principle of α-γ coactivation.
Table 2.2 Classification of peripheral nerve afferent and efferent fibers
Receptor type | Name | Function |
---|---|---|
Mechanoreceptors | ||
Muscle and skeletal | Muscle spindle | Limb position and motion |
Golgi tendon organ | Muscle tension | |
Joint receptor | Joint tension and angle | |
Cutaneous and subcutaneous | Ruffini’s ending | Pressure |
Merkel’s disk | Pressure | |
Meissner’s corpuscle | Touch velocity (hairless skin), low frequency vibration (flutter) | |
Hair receptors | Tactile (hairy skin) | |
Pacinian corpuscle | Touch acceleration, high-frequency vibration | |
Thermoreceptors | C bare nerve endings | Warm |
Aδ myelinated | Cold | |
Nociceptors | Aδ myelinated | High pressure, thermal and mechanothermal |
C bare nerve endings | Thermal and mechanothermal, polymodal, tissue damage products |
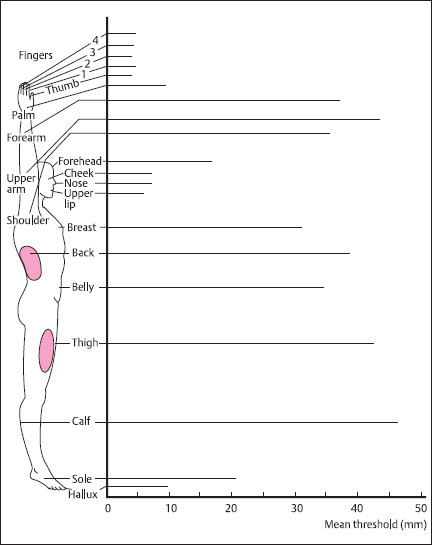
Fig. 2.6 Two-point discrimination thresholds for different regions of the body, measured as the smallest detectable separation distance between the tips of a calibrated compass. Thresholds vary widely over the body, being at their lowest (2mm) for the finger tips and highest for the forearm, legs, and back (40-50 mm). For selected regions, thresholds are proportional to the diameter of the receptive fields of individual afferents (shown in black). (From [1], with permission)
For the other proprioceptors, joint receptors are located in the connective tissue capsule and they respond to stretch of this tissue to signal joint pressure and angle. Golgi organs are found in the tendons and signal stretch resulting from muscle contraction. In terms of sensation, all these receptor types contribute to the sense of limb position and kinesthesia, along with information from cutaneous mechanoreceptors.
There are separate thermoreceptors for warm or cold stimuli, and like the skin mechanoreceptors they have punctate receptive fields, although they are bare nerve endings rather than encapsulated structures [13]. Warmth is mediated by receptors activated by a range of temperatures between approximately 32 °C and 45 °C, the discharge rate being proportional to temperature. Above 45 °C heat pain, rather than warmth, is perceived, due to the activity of thermal nociceptors, and within this range the discharge of warm receptors actually decreases. Cutaneous cold receptors are activated by temperatures from 1 °C to approximately 20 °C below ambient skin temperature, discharge frequency being roughly proportional to temperature difference. A sensory illusion called paradoxical cold occurs when a 45 °C hot stimulus is selectively applied to a cold fiber receptive field. The stimulus is perceived as cold (rather than warm or painfully hot, which would be the sensation when applied diffusely to the skin), and this coincides with an increased receptor discharge at these high temperatures.
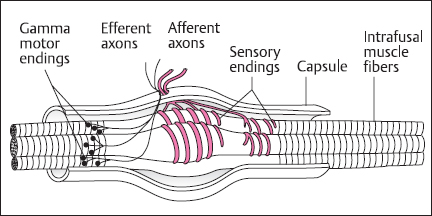
Fig. 2.7 Schematic of the muscle spindle. The main components of the spindle are the intrafusal muscle fibers, sensory afferent endings, and γ-motoneuron efferent fibers. The intrafusal fibers are devoid of contractile apparatus in the region of the afferent endings, although the ends are contractile and are innervated by the γ-motoneurons. The sensory endings are responsive to stretch of the intrafusal fibers. Contraction of the ends of the fibers alters spindle sensitivity. (From [l], with permission)
Nociceptors are also bare nerve endings and respond selectively to stimuli that are sufficiently intense that they could damage tissue, and to chemicals released as a result of tissue damage [14]. Thermal nociceptors respond selectively to extreme heat or cold; mechanical nociceptors are activated by strong mechanical stimulation, most effectively by sharp objects. Chemically sensitive, mechanically insensitive nociceptors respond to a variety of agents including K+, extremes of pH, and neuroactive substances such as histamine, bradykinin, and prostanoids, as well as various irritants. Polymodal nociceptors respond to combinations of mechanical, thermal, and chemical stimulation.
Pain is an unpleasant sensation triggered by tissue damage, real or potential. The high emotive content lends subjectivity to the experience, and simple stimulation of nociceptors does not necessarily lead to pain sensation under all circumstances: there are descending pathways that influence pain transmission. The physiological activation of nociceptors, though, usually gives rise to pricking, burning, aching, and stinging sensations. When the skin is damaged, the initial sensation is conveyed by Aδ fibers. C fibers are more important for the longer-lasting perception that outlives the stimulus. However, pain can also result from neural damage and changes in neural circuitry, and does not necessarily require activation of nociceptors. This is clearly seen in the case of phantom limb pain after surgical limb amputation. Such neuropathic pain following peripheral nerve injury and degeneration/regeneration can be caused by alterations in the balance of inputs to the spinal cord sensory neurons. Thus, large myelinated neurons may regenerate better than C fibers, so that spinal cord neurons that once had predominantly nociceptive input could now be dominated by Aβ touch fibers, although the central connection would remain appropriate for pain transmission. Such a phenomenon may underlie allodynia, where previously innocuous stimuli become severely painful [2,15].
Sensory Input to the Spinal Cord
The cell bodies of sensory neurons are located in the dorsal root ganglia. These bipolar neurons have a relatively long peripheral axon branch, and those in the dorsal column-medial lemniscal pathway also have an extensive central axonal projection. Thus, the cell bodies have a considerable task to supply nutrients and materials to maintain axonal function. This consideration, combined with the potential effects of diabetes on dorsal root ganglion microenvironment, could contribute to a relative vulnerability of sensory neurons, which would affect both peripheral and central projections. Evidence for involvement of central axons is seen in a reduction of spinal cord cross-sectional area, determined by magnetic resonance imaging in diabetic patients with distal symmetrical polyneuropathy [16].
The architecture of the spinal input reflects the segmental organization of embryonic development. As the embryo grows and expands, the developing skin carries its segmentally derived innervation with it. Thus, a single area of skin, a dermatome, is supplied by axons from a single dorsal root ganglion [1,2]. This dermatomal organization is shown in Figure 2.8. However, peripheral nerves themselves contain axons from several spinal roots, and different nerves can contain axons from the same root, so the relationship between peripheral nerve trunk and dermatome is complex.
In addition to a central projection, there are also local connections in the spinal cord for neurons that project in the dorsal column-medial lemniscal pathway. Second-order neurons for the spinothalamic projection are also located in the dorsal horn of the spinal cord [17]. The synaptic connections for the different sensory fiber types are made in different layers of the dorsal horn. Thus, Aδ fibers synapse primarily in laminae I and V; C fibers connect mainly in laminae II and I; Aβ input goes to lamina V neurons.
Control of Pain Transmission
Pain transmission from the spinal cord can be modified by nonpainful sensory input as well as by activation of descending pathways from various brain nuclei. Painful sensations evoked by activity of nociceptors (Aδ and C fibers) can be decreased by simultaneous stimulation of low-threshold mechanoreceptors, which may underlie the pain-reducing effects of rubbing of the skin and transcutaneous and dorsal column electrical stimulation. This derives from the gate theory of pain[18], according to which pain results from the balance of activity in nociceptive and nonnociceptive afferent fibers. Thus, nonnociceptive Aβ fiber activity “closes” the central transmission gate whereas nociceptive activity “opens” it. The detailed spinal cord circuitry responsible for this effect is not known, but neurons in lamina V receive convergent input from Aβ. Aδ, and C fiber afferents. Furthermore, Aβ fiber activity can suppress firing of lamina V neurons via inhibitory interneurons in lamina II.
Spinal pain transmission is also modulated by descending inputs [19,20]. Neurons in the periaqueductal gray matter of the midbrain make connections with the cells in the rostroventral medulla, particularly in the nucleus raphe magnus. These in turn project to the spinal cord and make inhibitory connections with neurons in laminae I, II, and IV. Thus, electrical stimulation of periaqueductal gray or raphe nuclei inhibits dorsal horn neurons, including those giving rise to the spinothalamic tract, providing profound analgesia. There are several important neurotransmitter systems involved. The periaqueductal gray area has a very high density of opioid receptors. The raphe nucleus contains many serotonergic neurons. Another descending pathway, from the midbrain locus ceruleus, is noradrenergic. The serotonergic and noradrenergic fibers stimulate dorsal horn interneurons that release the endogenous opiate neurotransmitter enkephalin to pre- and postsynaptically inhibit spinothalamic tract projection neurons.
Motor Output and Sensorimotor Integration in the Spinal Cord
The final common motor pathway to the skeletal muscles is via the motoneurons, whose axons make up a substantial proportion of the myelinated fiber population of peripheral nerve. The major motor output of the spinal cord comes from the α-motoneurons, which directly stimulate skeletal muscle force production by synaptic activation at the motor end plate. The other cord output, from γ-motoneurons, exerts an indirect influence on muscle tension by controlling muscle spindle sensitivity and dynamic range, which consequently affects reflex activation of α-motoneurons.
The basic element of motor control is called the motor unit, which comprises an α-motoneuron together with all of the muscle fibers that it innervates [21]. The collection of α-motoneurons that innervates a single skeletal muscle is termed the motor unit pool of that muscle. The size of the motor units varies greatly. For muscles involved in high-precision movements such as those of the digits or face, the number of muscle fibers may be only 10. For large muscles of the trunk and limbs there may be 3000-4000 fibers per motor unit. These fibers may be distributed over the entire area of the muscle, so the territory of a single motor unit may be considerable. All muscle fibers in an individual motor unit are biochemically, histochemically, and physiologically identical, indicating the determination of muscle fiber properties by their innervation. In terms of muscle and contractile properties, there are three motor unit types. Those based on type I muscle fibers are characterized by relatively slow contraction speeds, reliance on aerobic energy metabolism, and a profuse capillary supply-features that confer extreme fatigue resistance. These units are active for much of the time, being involved in postural control, and are preferentially recruited by muscle spindle afferent input to the spinal cord. In contrast, type IIB motor units have fast contraction times, rely on anaerobic metabolism, have relatively poor vascular supply, and fatigue rapidly. They are preferentially recruited for large, fast movements such as limb withdrawal from a painful stimulus. Type IIA units are somewhere in between: the muscle fibers are fast contracting, well supplied with capillaries, have both aerobic and anaerobic energy production, and are moderately fatigue-resistant.
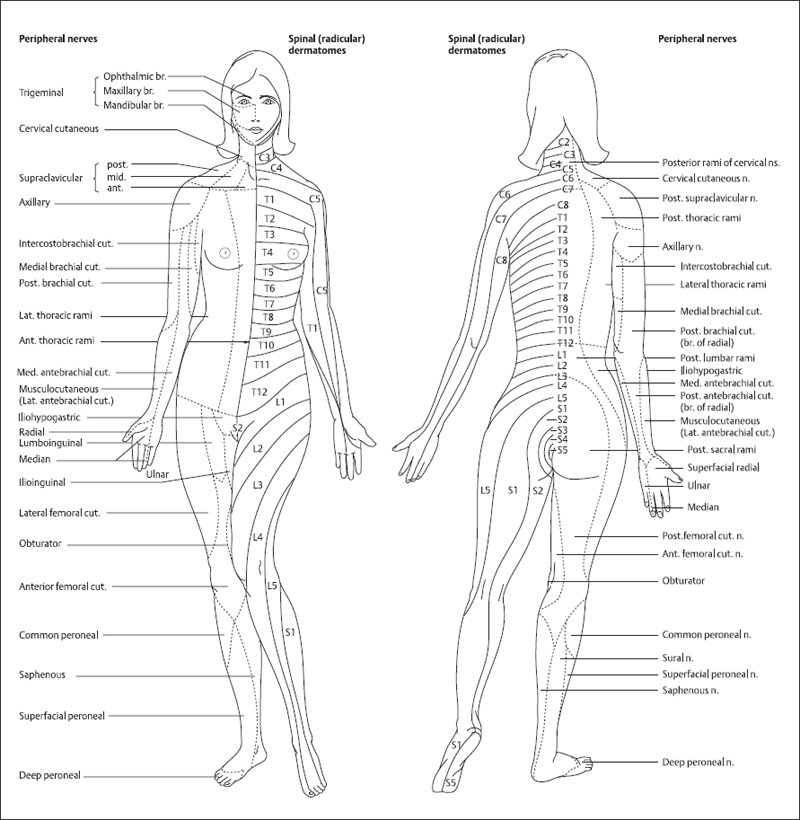
Fig. 2.8 Distribution of dermatomes and peripheral nerve patterns. Mapping of sensory innervation of the skin by the dorsal roots is shown on the left of the subject: cervical (C1-C7), thoracic (T1-T12), lumbar (L1-L5), and sacral (S1-S5). There is no dorsal root at C1, only a ventral (motor) root. The innervation patterns of peripheral nerves are shown for comparison on the left. Individual peripheral nerves have fibers that arise from several adjacent dorsal roots, leading to rather larger fields of innervation and overlap in the area innervated by each segment. (From [2], with permission)
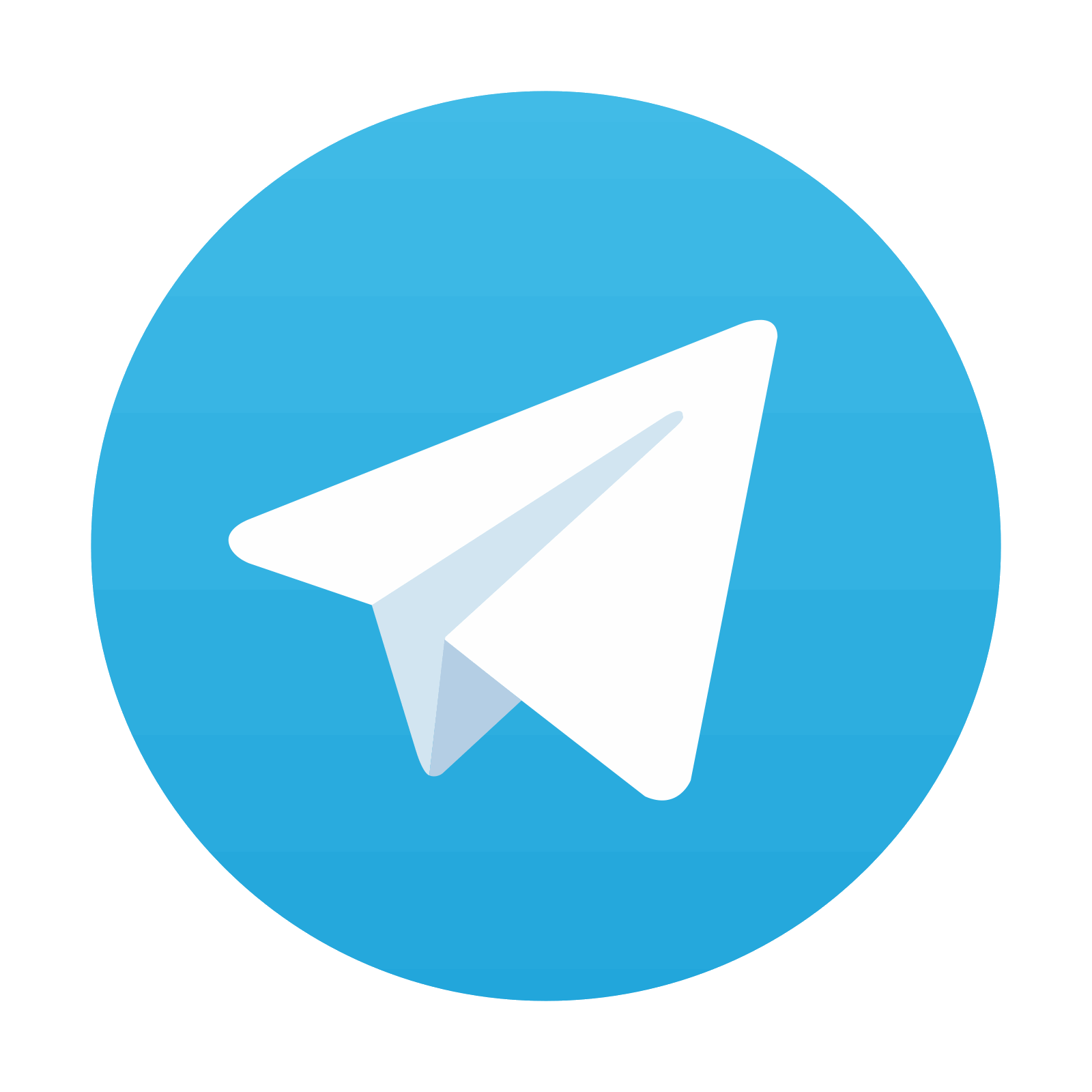
Stay updated, free articles. Join our Telegram channel
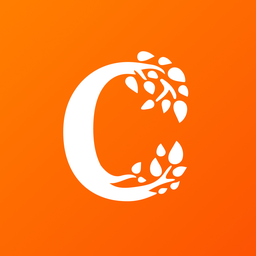
Full access? Get Clinical Tree
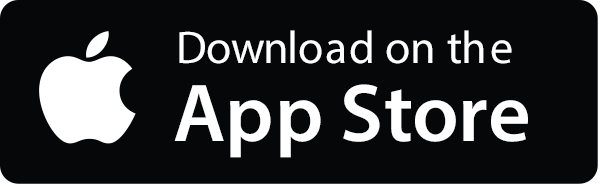
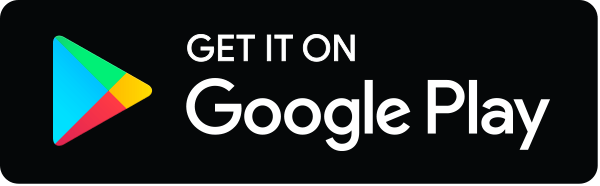