Licensed RBC additive solutions
Constituents (mM)
SAGM
AS-1
AS-3
AS-5
AS-7
MAP
PAGGSM
NaCl
150
154
70
150
85
72
NaHCO3
26
Na2HPO4
12
16
NaH2PO4
23
6
8
Citric acid
2
1
Na-citrate
23
5
Adenine
1.25
2
2
2.2
2
1.5
1.4
Guanosine
1.4
Glucose
45
111
55
45
80
40
47
Mannitol
30
41
45.5
55
80
55
pH
5.7
5.5
5.8
5.5
8.5
5.7
5.7
FDA licensed
No
Yes
Yes
Yes
Yes
No
No
Approved shelf life (d)
42
42
42
42
42a
42
49
The FDA and foreign counterparts allow for RBCs to be stored for a maximum of 42 days (49 days for PAGGS-M and 56 days for AS-7 in Europe) [11]; however, no clinical study assessing the clinical safety of this somewhat arbitrary decision was ever conducted. The expiration date of RBC units are mainly based on post transfusion recovery studies, which measure the quantity of chromium radiolabeled RBCs remaining in the peripheral circulation 24 h after a dose of autologous RBCs is reinfused into healthy subjects on the last day of storage [12]. Hemolysis levels in the bag are also taken into consideration [13]. Earlier FDA standards in the 1970s set 70 % as an acceptable 24-h post transfusion RBC recovery. This level was decided for concern that any lower recovery could result in hemoglobinuria which would be confused with an immunologic reaction to incompatible RBCs [12]. The FDA standards were arbitrarily updated in 1985 to a minimum mean recovery of 75 % of radiolabeled RBCs at 24 h [12]. Additionally, in the USA and Europe, hemolysis must be less than 1.0 and 0.8 %, respectively, at the end of storage (with 95 % confidence that at least 95 % of the population meets or exceeds the specification) [13].
Blood banking has come a long way since the first blood banks during World War I. The development of additive solutions allowed for longer storage of RBC units [5]. Due to the challenges of providing RBCs with a limited supply, there is pressure to increase the shelf life of RBC units. However this must be balanced with the RBC storage lesion that results from prolonged refrigerator storage of RBCs. The storage lesion is “a series of biochemical and biomechanical changes in the RBC and storage media during ex vivo preservation that reduce RBC survival and function” [14]. The storage lesion has been studied for decades. The potential contributors to the storage lesion include changes in RBC morphology and deformability, reductions in 2,3-DPG, ATP, pH, reduced glutathione, membrane expression of CD47, as well as membrane loss and increased formation of microvesicles, oxidation of cellular lipids and proteins, phosphatidylserine exposure, supernatant lipid accumulation and desialylation of RBCs (see Fig. 9.1). The underlying mechanisms of these contributors to the storage lesion will be further described below.


Fig. 9.1
Features of the RBC storage lesion . The biochemical and biomechanical changes occur in the storage media or RBC additive solution, RBC membrane, and RBC. Hemoglobin (Hb), phosphatidylserine (PS)
Potential Contributors to the RBC Storage Lesion
RBC Morphology and Deformability
During storage, red blood cells undergo a predictable morphologic change that can be visualized by electron microscopy (see Fig. 9.2). Some of these shape changes are reversible following transfusion, while some changes are irreversible. RBCs undergo a transition from the normal biconcave disk shape to reversibly deformed echinocytes, which have projections extending from the surface resembling a spiked ball. Eventually, the changes become irreversible as the RBCs become spheroechinocytic. During the first week of storage, discocytes predominate. By day 21 of storage, nearly half of the RBCs are abnormally echinocytically shaped, and by day 42 over 76 % of RBCs are abnormally shaped with a substantial percentage of irreversibly changed spheroechinocytes [15]. These morphologic transitions are accompanied by a decrease in RBC membrane deformability [15, 16]. The relationship between abnormally shaped RBC and RBC deformability is unclear. However, at early stages of storage, normal appearing biconcave disks exhibited reduced deformability [17] suggesting that changes in membrane deformability may precede the alterations in cell shape. Using a microbead sorting device to recapitulate the splenic microcirculation, older stored RBCs were shown to have a significant increase in elongation index and retention rate. This suggests that older stored RBCs are less deformable and are more likely to be retained and destroyed by the spleen [18]. The full implications of the changes in morphologic and rheological properties of RBCs during storage remain to be fully determined.


Fig. 9.2
Scanning electron microscopic image of RBCs during storage. (a) On the 5th day of storage, discocytes predominate and only a few irreversibly changed RBC can be seen. (b) On the 14th day of storage, numerous echinocytes and spheroechinocytes are observed. (c) By the 42nd day of storage, spheroechinocytes and degenerated forms predominate among the irreversibly changed RBCs. (Reproduced from Berezina et al. 2002)
2,3-Diphosphoglycerate (DPG)
2,3-DPG is the major allosteric modifier of oxygen affinity and causes a right shift in the oxygen-hemoglobin dissociation curve thereby enhancing off loading of oxygen. A decrease in RBC levels of 2,3 DPG has been observed with RBC storage with nearly undetectable levels by 2–3 weeks of storage [19]. This change impairs oxygen release to tissues following transfusion; however, RBC 2,3-DPG levels regenerate relatively quickly and levels have been shown to increase by 25–30, 50, and 100 % by 1 h, 24 h, and 3 days following transfusion, respectively [20]. In addition to decreased 2,3-DPG levels , metabolomics studies suggest decreases in ATP, pH, and reduced glutathione levels accompanied by increases in oxidized glutathione, lactate, NADPH, and oxidation of membrane proteins and lipids during prolonged storage [16, 21].
Adenosine Triphosphate (ATP) , pH, Lactate
Multiple studies observe a decrease in pH, a decrease in ATP, and an increase in lactate during storage [16, 21, 22]. Experimental ATP depletion results in morphologic changes in RBCs from their normal discoid shape to a crenated one and then eventually to smooth spheres after 6 h. These changes are similar to those observed during RBC storage. Furthermore, restoration of RBC ATP levels results in return of their normal biconcave disk shape [23]. This demonstrates the importance of ATP in normal RBC morphology. However, although parallels can be drawn between these experiments involving rapid ATP depletion and then restoration, the depletion of ATP in RBCs during storage is a much more gradual process. Higher ATP levels in stored blood from different storage media has little effect on the viability of RBCs when transfused, suggesting that higher ATP levels may not be critical for RBC survival [24]. Additionally, despite ATP restoration to normal levels, a number of irreversible spherocytes are still present [14].
Glutathione and NADPH
Oxidation occurs in RBCs due to transported oxygen and the reactive iron present in hemoglobin [25]. Oxidizing radicals such as superoxide anions, hydrogen peroxide, and hydroxyl radicals are generated during RBC storage [26]. In stored RBCs these oxidizing radicals modify both lipids and proteins, damaging RBC membrane integrity. Antioxidants are available to counteract the destructive effects of this oxidation. The primary antioxidant defense system in stored RBCs is reduced glutathione and glutathione peroxidase. During storage, there is a significant decline in both reduced glutathione and glutathione peroxidase levels with a concomitant rise in oxidized glutathione [21, 26]. These changes are indicators of oxidative stress during storage.
Metabolomics studies of RBCs during refrigerated storage reveal a shift towards the oxidative phase of the pentose phosphate pathway [27]. This shift is likely a response to the increase in oxidative stress and accumulation of reactive oxygen species observed during storage. Thus, NADPH levels increase during storage in line with the switch towards the pentose phosphate pathway [21]. This potentially allows the RBC to maintain oxidation–reduction balance and protect itself against oxidative injury [28].
Oxidation of Cellular Lipids and Proteins
The generation of oxidizing radicals results in oxidative damage to the RBC membrane lipids and proteins. There is both a loss of membrane lipids as well as increase in lipid peroxidation with RBC storage [21, 26]. Peroxidation of RBC membrane phospholipids results in the formation of malondialdehyde, a highly reactive molecule that interacts with RBC membrane phospholipids and proteins and impairs their function. Malondialdehyde has been observed to increase progressively during storage [21, 26]. Oxidative damage also modifies membrane proteins of RBCs. Oxidation further induces aggregation of proteins, introducing carbonyl groups to specific residues of polypeptide chains. During RBC storage, carbonylation of band 4.1 protein, an important cytoskeletal protein, increases significantly [26]. Additionally, protein aggregation of band 3 and a shift of small and large band 3 oligomers to large aggregates is observed [29]. Band 3, the anion exchanger 1, is a membrane receptor and transporter protein with a number of important roles including maintenance of the oxygen transport system, docking site for glycolytic enzymes, and structural protein in the cytoskeletal network [30].
Glycolytic enzymes form complexes with band 3 on the RBC membrane. When glycolytic enzymes are bound to band 3 their function is inhibited, when they are released and free in the cytoplasm, their activity is restored. During blood bank storage, in response to oxidative stress, these glycolytic enzymes are increasingly bound to band 3 and thus inhibited. Thus, this may explain the mechanism for the shift towards the oxidative phase of the pentose phosphate pathway, resulting in increased production of NADPH [31]. Finally, band 3 aggregation occurs both in vitro in the storage lesion as well as in vivo associated with aging RBCs in circulation and potentially serves as a membrane neoantigen for IgG to bind and mediate phagocytosis by Kupffer cells [30].
Membrane Loss and Microvesicles
Stored RBCs undergo membrane oxidation, damage, and exocytosis to form microvesicles (50–100 nm), which contain a substantial amount of hemoglobin. Some studies demonstrate that a small proportion of microvesicles are already evident at 4 days, but the percentage of microvesicles in packed RBC units increases dramatically with storage duration [32]. These microvesicles are prothrombotic, proinflammatory, and immunogenic and therefore are a potential source of adverse post-transfusion effects [33].
Supernatant Lipid Accumulation
During RBC storage, both nonpolar lipids and lysophosphatidylcholines accumulate in the supernatant. These lipids can prime polymorphonuclear cells and mediate acute lung injury in vivo [34]. The nature of the lipids responsible for this effect is different for leukoreduced and non-leukoreduced RBC units. Leukoreduced RBCs contain mostly the nonpolar lipids with arachidonic acid and 5-, 12-, 15-hydroxyeicostetraenoic acid (HETE) responsible for the priming activity; non-leukoreduced units also accumulate lysophosphatidylcholines, which can also mediate this potentially deleterious effect [34].
Phosphatidylserine
The RBC plasma membrane, like other cell membranes, is composed of a phospholipid bilayer. This bilayer demonstrates a distinct asymmetry with choline-containing lipids, phosphatidylcholine and sphingomyelin, predominately in the outer leaflet, while the aminophospholipids, phosphatidylethanolamine and phosphatidylserine (PS) are located mainly in the inner leaflet of erythrocytes [35]. This asymmetric distribution is tightly regulated by the enzymes flippase and scramblase. Flippase is an ATP-dependent aminophospholipid translocase that transports PS from the outer to inner leaflet; conversely, phospholipid scramblase is responsible for the transport of PS from the outer to inner leaflet. The maintenance of the phospholipid asymmetry is essential for cell survival. Specifically, PS exposure is an apoptotic signal and enhances pro-coagulant activity as it serves as a negatively charged surface for the coagulation cascade. RBC storage results in an increase in PS exposure in some [35], but not all studies [16]. However, although PS exposure may not occur during cold storage in vitro [16], it is difficult to assess whether stored RBCs are more prone to expose PS on their external membrane surface once transfused. Furthermore, flippase activity declines with storage. Flippase activity is modulated by ATP levels and intracellular pH; notably, both ATP levels and pH decline with RBC storage. Flippase activity can be restored to those seen in freshly isolated RBCs through experimental manipulation restoring ATP levels and pH [35]. These findings suggest that incubation in a high pH rejuvenation solution of inosine, phosphate, pyruvate, and adenine can rejuvenate stored RBCs and improve post-transfusion recovery [36].
Desialylation of RBCs
Exposure of the penultimate β-galactosyl residues as a result of desialylation of membrane glycoconjugates has been demonstrated to promote phagocytosis of senescent RBCs in circulation [37]. During storage of non-leukoreduced RBC units, RBCs are desialylated leading to exposure of terminal β-galactosyl residues. However, leukoreduction of RBC units dramatically reduces this desialylation. This finding, along with the accumulation of lysophosphatidylcholines in non-leukoreduced RBC units, which could potentially mediate acute lung injury [34], demonstrate some of the benefits of leukoreducing RBC units.
Decreased Membrane Expression of CD47
CD47, or integrin associated protein, is a transmembrane protein found on RBCs. It has been shown to have an important role as a self-recognition marker, acting as a “don’t eat me” signal, and thus protects circulating RBCs from destruction by macrophages [38]. CD47 interacts with signal regulatory protein alpha (SIRPα) receptors found on macrophages, which causes inhibition of macrophage activation and thus survival of circulating RBCs [39]. CD47 antigen expression decreases on RBCs during storage, potentially enhancing their phagocytosis by macrophages. Additionally, with the loss of CD47 from the RBC surface, an increase in CD47 is observed in the supernatant of the RBC units. This extracellular CD47 may mediate a deleterious effect on the host during transfusion by binding and interfering with signal transduction pathways [38].
Clinical Consequences of Transfusion of Stored RBCs
RBC Hemolysis
Controversy exists as to whether transfusion of older, stored RBCs has negative clinical implications. Furthermore, the mechanism(s) responsible for adverse effects has yet to be determined. It is well-accepted that with increasing storage there is decreasing recovery of RBCs following transfusion leading to the FDA criteria that on average >75 % of RBCs remain in the circulation 24-h following transfusion. Thus, up to 25 % of transfused RBCs can be cleared from the circulation. Two leading hypotheses regarding the adverse effects of the storage lesion relate to hemolysis and differ in terms of the relative contribution of intravascular versus extravascular hemolysis. Hemolysis is defined as the destruction or removal of old or damaged RBCs from the circulation [40]. Certain types of damage result in intravascular hemolysis, in which the RBCs are destroyed within the circulation, releasing free hemoglobin and the remaining RBC contents. Extravascular hemolysis occurs by phagocytosis in the monocyte–macrophage system of organs such as the liver and spleen, which are considered the primary site of RBC removal from the circulation [41, 42]. Finally, to the extent that clinically relevant adverse effects of transfusion exist, it is likely that a combination of intravascular and extravascular hemolysis, along with other elements of the storage lesion described above, is responsible for these effects (Fig. 9.3).


Fig. 9.3
Intravascular and extravascular hemolytic pathways . Intravascular hemolysis results in the formation of free hemoglobin (Hb) and heme, which bind haptoglobin and hemopexin, respectively, and are delivered to the liver for degradation. Extravascular hemolysis occurs within reticuloendothelial macrophages. The breakdown of free Hb results in the production of iron, carbon monoxide, and biliverdin. Outside of the macrophages, free iron binds transferrin to be safely transported in the bloodstream. If these systems are saturated, non-transferrin-bound iron (NTBI) is produced and nitric oxide (NO) is consumed. The production of NTBI, free hemoglobin (Hb), and heme can lead to the listed consequences, contributing to organ dysfunction and tissue ischemia
Intravascular Hemolysis
Transfusion of older, stored RBCs is associated with increased plasma free hemoglobin and pulmonary artery pressure in recipients [43]. During intravascular hemolysis, the toxic compounds, hemoglobin and heme, are released into the circulation. Because of the toxicity of these compounds, mammals are equipped with efficient but saturable systems to clear them from the circulation. Haptoglobin and hemopexin are the most important scavenger systems.
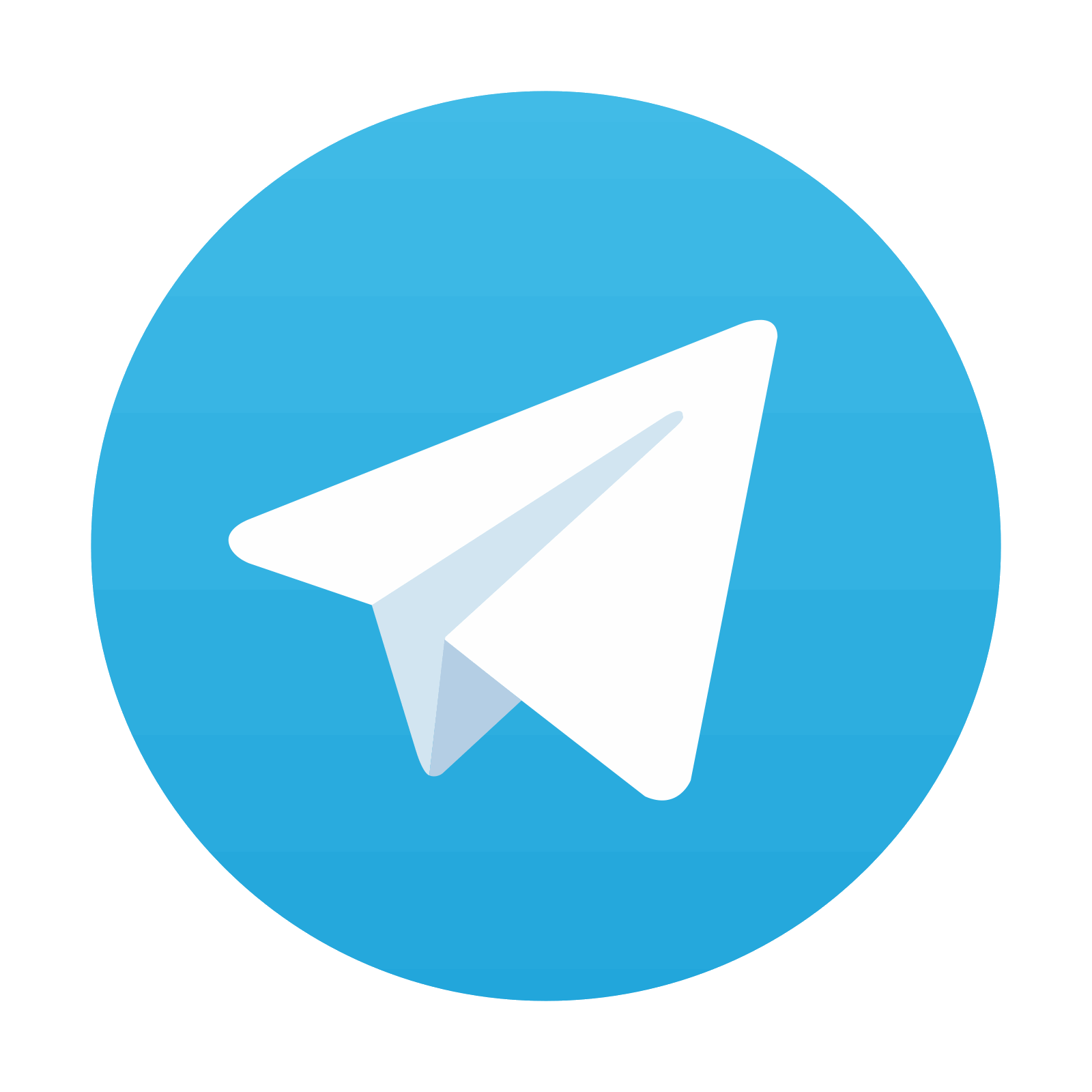
Stay updated, free articles. Join our Telegram channel
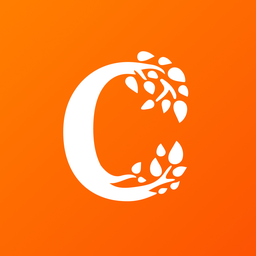
Full access? Get Clinical Tree
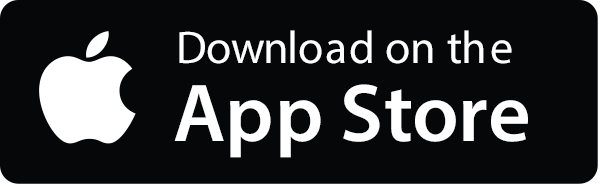
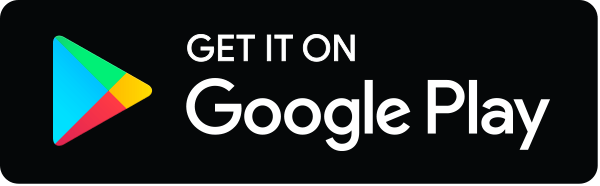