Fig. 14.1
Stem cells as a promising tool addressing generation of beta-like cells/insulin-secreting cells as well as immunomodulation
14.5 Early Attempts for In Vitro Beta-Cell Differentiation
Stimulation of beta-cell generation or generation of cells secreting insulin, for the treatment of IDDM, is attractive because it directly addresses the major functional deficiency underlying the disease. For IDDM therapy, it is not clear whether it will be desirable to produce only beta-cells—the islet cells that manufacture insulin—or whether other types of pancreatic islet cells are also necessary. Studies by Bernat Soria and colleagues indicate that isolated beta-cells—those cultured in the absence of the other types of islet cells—are less responsive to changes in glucose concentration, than intact islet clusters made up of all islet cell types. Islet cell clusters typically respond to higher-than-normal concentrations of glucose by releasing insulin in two phases: a quick release of high concentrations of insulin and a slower release of lower concentrations of insulin. In this manner the beta-cells can fine-tune their response to glucose. Extremely high concentrations of glucose may require that more insulin be released quickly, while intermediate concentrations of glucose can be handled by a balance of quickly and slowly released insulin.
Isolated beta-cells, as well as islet clusters with lower-than-normal amounts of non-beta-cells, do not release insulin in this biphasic manner. Instead insulin is released in an all-or-nothing manner, with no fine-tuning for intermediate concentrations of glucose in the blood (Soria et al. 1996; Bosco and Meda 1997). Therefore, many researchers believe that it will be preferable to develop a system, in which stem or precursor cell types can be cultured to produce all the cells of the islet cluster. A list of different sources of stem cells and their advantages and disadvantages is mentioned here (Table 14.1).
Table 14.1
A list of the sources of stem cells. Their advantages and disadvantages
Stem cell type | Description | Advantages | Disadvantages |
---|---|---|---|
Embryonic | Cells from human blastocysts | Pluripotent | Requires embryo destruction |
Fetal stem cells | Cells from gonads of aborted fetuses | Multipotent | Requires destruction of weeks old fetus |
Umbilical cord stem cells | Cells from the umbilical cord blood of newborns | Multipotent/pluripotent? | Low frequency of stem cells |
Placenta-derived stem cells | Cells from the placenta of newborns | Multipotent/pluripotent? | Low frequency (but higher than cord blood) |
Adult stem cells | Cells from adult tissues | Multipotent | Very low frequency |
Induced pluripotent stem (iPS) cells | Cells from adult tissues reprogrammed to pluripotency | Pluripotent | Not patentable |
14.6 Embryonic Stem Cells Experience
The discovery of methods to isolate and grow human-Embryonic Stem Cells (ESCs) in 1998 renewed the hopes of researchers, clinicians and diabetes patients and their families that a cure for IDDM and perhaps non-IDDM as well may be within striking distance. In theory, ESC could be cultivated and coaxed into developing the insulin-producing islet cells of the pancreas. With a ready supply of cultured stem cells at hand, the theory is that a line of ESC could be grown up as needed, for anyone requiring a transplant. The cells could be engineered to avoid immune rejection. Before transplantation, they could be placed into non-immunogenic material, so that they would not be rejected, and the patient would avoid the devastating effects of immunosuppressant drugs.
There is also some evidence that differentiated cells derived from ESCs might be less likely to cause immune rejection. Although having a replenishable supply of insulin-producing cells for transplant into humans may be a long way off, researchers have been making remarkable progress in their quest for it. While some researchers have pursued the ESC, others have focused on insulin-producing precursor cells that naturally occur in adult and fetal tissues.
In Spain it was reported using mouse ESCs that were engineered to allow to select for cells that were differentiating into insulin-producing cells (Soria et al. 2000). Bernat Soria and his colleagues added DNA containing part of the insulin gene to ESC from mice. The insulin gene was linked to another gene that rendered the mice resistant to an antibiotic drug. By growing the cells in the presence of an antibiotic, only those cells that were activating the insulin promoter were able to survive. The cells were cloned and then cultured under varying conditions. Cells cultured in the presence of low concentrations of glucose differentiated and were able to respond to changes in glucose concentration by increasing insulin secretion nearly sevenfold.
The researchers then implanted the cells into the spleens of diabetic mice and found that symptoms of diabetes were reversed. Manfred Ruediger of Cardion, Inc., in Erkrath, Germany, is using the approach developed by Soria and his colleagues to develop insulin-producing human cells derived from ESC. The non-insulin-producing cells will be killed off, and only insulin-producing cells should survive. This is important in ensuring that undifferentiated cells are not implanted that could give rise to tumors. However, some believe that it will be important to engineer systems in which all the components of a functioning pancreatic islet are allowed to develop.
Ron McKay and his colleagues described a series of experiments, in which they induced mouse ESC to differentiate into insulin-secreting structures, that resembled pancreatic islets (Lumelsky et al. 2001). McKay and his colleagues started with ESC and let them form embryoid bodies, and selected a population of cells from the embryoid bodies that expressed the neural marker nestin. Using a sophisticated five-stage culturing technique, they were able to induce the cells to form islet-like clusters that resembled those found in native pancreatic islets. The cells responded to normal glucose concentrations by secreting insulin, although insulin amounts were lower than those by normal islet cells. When the cells were injected into diabetic mice, they survived, although they did not reverse the symptoms of diabetes.
According to McKay, this system is unique in that the ESCs form a functioning pancreatic islet, complete with all the major cell types. The cells assemble into islet-like structures that contain another layer, which contains neurons, and were similar to intact islets from the pancreas. Several research groups are trying to apply McKay’s results with mice, to induce human ESC to differentiate into insulin-producing islets. Melton, Nissim Benvinisty of the Hebrew University in Jerusalem, and Josef Itskovitz-Eldor of the Technion in Haifa, Israel, reported that human ESC could be manipulated in culture to express the Pdx-1 gene, a gene that controls insulin transcription (Schuldiner et al. 2000; Melton 2006).
In these experiments, cultured human ESCs were allowed to spontaneously form embryoid bodies. The embryoid bodies were then treated with various growth factors, including nerve growth factor. They found that both untreated embryoid bodies and those treated with nerve growth factor expressed Pdx-1. ESC prior to formation of the aggregated embryoid bodies did not express Pdx-1. Because expression of the Pdx-1 gene is associated with the formation of beta-islet cells, these results suggest that such cells may be one of the types that spontaneously differentiate in the embryoid bodies. They think that nerve growth factor may be one of the key signals, for inducing the differentiation of beta-islet cells, and can be exploited in the laboratory.
Complementing these findings is work done by Jon Odorico of the University of Wisconsin in Madison, using human ESC of the same source. In preliminary findings, he had shown that human ESC can differentiate and express the insulin gene. Itskovitz-Eldor and his Technion colleagues further characterized insulin-producing cells in embryoid bodies (Assady et al. 2001). They found that ESCs that were allowed to spontaneously form embryoid bodies contained a significant percentage of cells that express insulin. Based on the binding of antibodies to the insulin protein, Itskovitz-Eldor estimated that 1–3 % of the cells in embryoid bodies are insulin-producing beta-islet cells.
The researchers also found that cells in the embryoid bodies express Glut-2 and islet-specific glucokinase, genes important for beta-cell function and insulin secretion. Although they did not measure a time-dependent response to glucose, they did find that cells cultured in the presence of glucose secrete insulin into the culture medium. They concluded that embryoid bodies contain a subset that appear to function as beta-cells, and that the refining of culture conditions may soon yield a viable method for inducing the differentiation of beta-cells and, possibly, pancreatic islets.
14.6.1 Problem with ESC Research
According to many stem cell researchers, ESCs are the preferred stem cells for cell-based therapies. Although they tend be more versatile than adult stem cells, other sources have proven to be just as versatile (Kögler et al. 2004). The same properties that make ESC so versatile also make them unusable for therapy. Unless completely differentiated prior to use in patients, they will migrate throughout the body to produce tumors. Experiments performed in mice and rats have shown that spontaneous tumor formation is a persistent problem (Bjorklund et al. 2002; Carson et al. 2006).
Maintaining and growing ESC lines have also been problematic. Some of these lines may become mutated, making them unusable in patients (Draper et al. 2004; Cowan et al. 2004). The main problem with ESC research is tissue incompatibility (Phimister and Drazen 2004). In contrast to ESC technologies, adult stem cells have been used to treat some of the diseases, with the list growing every year. Pursuing this technology would eliminate the tissue rejection problems associated with ESC and the high cost associated with therapeutic cloning.
14.7 Fetal Tissue as Source for Islet Cells
Several groups are investigating the use of fetal tissue as a potential source of islet progenitor cells. For example, using mice, researchers have compared the insulin content of implants from several sources of stem cells—fresh human fetal pancreatic tissue, purified human islets, and cultured islet tissue (Beattie et al. 1997). They found that insulin content was initially higher in the fresh tissue and purified islets. However, with time, insulin concentration decreased in the whole tissue grafts, while it remained the same in the purified islet grafts. When cultured islets were implanted, however, their insulin content increased over the course of 3 months. The researchers concluded that precursor cells within the cultured islets were able to proliferate (continue to replicate) and differentiate (specialize) into functioning islet tissue, but that the purified islet cells (already differentiated) could not further proliferate when grafted. Importantly, the researchers found, however, that it was also difficult to expand cultures of fetal islet progenitor cells in culture.
Although precedents exist for the clinical use of human stem cells, there is considerable reluctance to proceed with clinical trials involving cells derived from embryonic and fetal sources. Alternative sources are listed here (Table 14.2).
Table 14.2
Alternative sources of stem cells for generation of beta/islet-like cells
Sources | Scientific studies (in vivo/in vitro study) |
---|---|
Embryonic stem cells | Generate embryoid bodies that contain cells with a B-cell-like phenotype with overexpression of PAX4, PDX1, or NKX6, during differentiation in human ESCs, while in case of Murine ESCs overexpression of insulin. Failure of study due to development of tumors in animal |
Bone marrow-derived stem cells | BMSCs in regenerative processes in vivo remain the subject of study because infusion of bone marrow cells can restore chemically induced diabetes in mice. Notably, however, it has not been indisputably shown that BMSCs differentiate into beta-cells |
Placenta-derived multipotent stem cells | Differentiation into insulin-positive cells which functionally secrete insulin in vitro Control blood glucose levels in vivo |
Liver stem cells | When transplanted into immunodeficient diabetic mice, transduced with human telomerase (hTERT) and then PDX-1 produced cells with considerable amounts of stored and secreted insulin and maintained euglycemia for prolonged periods |
Gastrointestinal adult stem cells | Transfected intestinal stem cells from the rat with genes encoding Pdx-1 and Isl-1, followed by exposure to the peptide betacellulin, which promotes pancreatic B-cell differentiation. The resultant cells made insulin and reduced glucose levels in vivo |
Adipose tissue-derived stem cell | During the proliferation period, the cells expressed stem cell markers nesting, ABCG2, SCF, Thy-1, and Isl-1 together with induction of insulin, glucagon, and somatostatin |
14.8 Adult Tissue as Source for Islet Cells
Adult stem cells such as BM and cord blood stem cells, which have been administered to many patients without adverse effects, are already recognized as being capable of differentiating into cells such as ISC. The production of insulin has already been demonstrated in animal models in which mesenchymal stem cells (MSC) were administered into mice. The use of adult stem cells to induce islet regeneration is also currently undergoing U.S. FDA approved clinical trials at the University of Miami. Additionally, results from numerous clinical studies involving the administration of BM stem cells by physicians outside of the U.S. have been very promising. One group in Argentina has reported that 85 % of type 2 diabetic patients who were treated with their own MSC were able to stop using insulin (Soria et al. 2005; Kaufman 2011; Leon-Quinto et al. 2004). Paracrine activity and capacity to reverse secondary complications are the other main characteristics of adult cells for their potential use (Leon-Quinto et al. 2004).
14.9 Pioneering Cell Therapy for IDDM
By the end of 2003, cell therapy for IDDM started being performed in humans, and reportedly the world’s first study was performed at the University of São Paulo—Brazil (Voltarelli et al 2007, 2008). The basic inclusion criteria were age between 12 and 35 years and a diagnosis of IDDM less than 6 weeks prior to inclusion, confirmed by positive serum levels of anti-GAD antibodies.
In the first stage of the protocol, called mobilization, a small dose of cyclophosphamide was administered intravenously to mobilize HSC from the BM to the peripheral blood, followed by daily subcutaneous injections of the granulocyte colony stimulating factor to proliferate circulating stem cells; these cells were then collected and frozen.
Ten to fifteen days later, in second phase, called the conditioning regimen, high dose immunosuppression was used with intravenous cyclophosphamide 200 mg/kg plus intravenous rabbit anti-thymocyte globulin 4.5 mg/kg, with the aim of “turning off” the immune system, mainly the peripheral T-cells that retain immunologic memory. Later, they intravenously reinfused the previously collected HSC, which do not have immunologic memory, to regenerate a new immune system that will not attack pancreatic beta-cells. The procedure was called “immunologic resetting” and no beta-cells were regenerated, but those not yet destroyed were preserved. They included only newly diagnosed patients—that is, patients who still have residual beta-cell mass to be preserved.
Up to December 2008, they performed this procedure in 23 patients: 17 men and 6 women aged 13–31 with an average body mass index of 19.7 kg/m2, glycemia level of 395.6 mg/dl, and glycosylated hemoglobin (HbA1c) level of 8.4 % at the time of diagnosis, and undergoing anti-glutamic acid decarboxylase (GAD) treatment ranging from 1.1 to 102 U/ml, and using an average dose of 0.4 UI/kg/day soon before starting the treatment.
All patients were recommended to be on a diet and to exercise regularly within a regimen of intensive insulin therapy and carbohydrate counting to try and achieve goals like: preprandial glycemia <120 mg/dL, postprandial glycemia <140 mg/dL, and HbA1c <7 %. Patients also received psychological guidance.
Of the 23 patients included, 20 remained free from insulin for some period. Of these, 12 have been continuously insulin-free since treatment, 8 became transiently insulin-free, and 3 maintained daily insulin doses. In the group of continuously insulin-free patients, most stopped daily insulin injections soon after stem cell infusion, and the mean period free from insulin was 31 months (ranging from 14 to 52 months). There was a significant reduction in HbA1c compared with pretreatment values, with all measures below 7 % during follow-up. In parallel, in this group of patients they observed important increases in mean C-peptide levels (0.8 nmol/L pretreatment vs. 2.9 nmol/L after 3 years; P <0.05).
Here three patients did not experience any period free from insulin. The limitations of the study, were risk of infections, elevated cost, need of an ultra-specialized team, requirement for patients to have been diagnosed no later than 6 weeks prior to treatment, and so on (Couri and Voltarelli 2009).
In 2006, a Chinese group from the University of Naijing started a similar protocol, with the difference that the stem cell infusion was 50 % into the peripheral vein and 50 % straight into the pancreas, through arterial catheterization. Of the five patients initially treated less than 3 months after diagnosis, four became insulin-independent (two of them only transiently) and one had a 50 % decrease in insulin dose. All 11 patients who had been diagnosed over 3 months before treatment kept using insulin.
In 2008, researchers at the Medical School of Ribeirao Preto—USP started studies in humans with IDDM using MSC. The protocol included BM biopsy under general anesthesia in first-degree relatives for the collection of MSC. These cells were sent to a laboratory to be stimulated to proliferate for a month, and were later infused into the patient through a gelatinous solution of approximately 100 ml with no use of chemotherapy. Two patients have been included in this protocol but no follow-up data has been published (Couri and Voltarelli 2009).
Another form of cell therapy is that performed in Argentina, China, and United States, using stem cells from the patient’s own BM (including a conglomerate of MSC and HSC), obtained in a bone marrow biopsy. While still under anesthesia, these cells are infused by arterial catheterization directly into the patient’s pancreas. This therapy was performed in 22 IDDM patients and in 31 non-IDDM patients. But the authors did not publish complete data.
14.10 MSC-Based Therapies
MSC are multipotent non-hematopoietic progenitor cells that are being explored as a promising new treatment for tissue regeneration. MSC can be derived from adult BM, fat, and several fetal tissues. In vitro, MSC have the capacity to differentiate into multiple mesodermal and non-mesodermal cell lineages. MSC have been shown to provide cytokine and growth factor support for expansion of HSC and ESC (Majumdar et al. 2000; Haynesworth et al. 1996; Cheng et al. 2000, 2003).
One of the most remarkable and least understood findings is the ability of MSC to migrate to sites of tissue injury (Pittenger and Martin 2004; Bittira et al. 2003; Mackenzie and Flake 2001; Wu et al. 2003). Besides, MSC possess immunosuppressive effects, by modulating the immune function of the major cell populations, involved in alloantigen recognition and elimination. The intriguing biology of MSC makes them strong candidates for cell-based therapy against various human diseases. Generation of ISC from MSC represents an attractive alternative. MSC from pancreas, BM, adipose tissue, umbilical cord blood (UCB), and cord tissue have the potential to differentiate into ISC by genetic modification and/or defined culture conditions in vitro. On the other hand, MSC are able to serve as a cellular vehicle for the expression of human insulin gene. Moreover, protein transduction technology could offer a novel approach for generating ISC from stem cells including MSC (Vija et al. 2009; Liu and Han 2008).
14.11 Pancreatic MSC to Treat Experimental Diabetes
There is evidence to suggest that pancreatic stem or progenitor cells reside within pancreatic duct cells, where they differentiate and migrate to form new islets during both organogenesis and regeneration. Pancreatic cells with the possibilities for generation of ISC are shown in Table 14.3. Ramiya et al. first described the generation of new islets from pancreatic duct epithelial cells in vitro, isolated from pre-diabetic adult non-obese diabetic (NOD) mice. These in vitro-grown islets contained alpha and delta cells, responded to in vitro glucose challenge and, once implanted into NOD mice, reversed IDDM (Ramiya et al. 2000).
Table 14.3
Pancreatic cells for generation of islet cells
Candidate Cells | Possible mechanisms |
---|---|
Ductal cells | Ductal cells: less differentiated; progenitor stage: islet cells |
β-cells | β-cells: less differentiated progenitor stage: islet cells OR β-cells: replication: increase β-cell mass |
Other progenitor cells | Progenitor cells (phenotypically less defined): islet cells |
Lu et al. demonstrated that transcription factor Pdx-1 plays an important role in the differentiation of pancreatic stem cells into pseudo-islet cells. Moreover, with the use of hepatocyte growth factor (HGF), neonatal pig pancreatic duct-derived cell monolayers could be induced to form three-dimensional islet-like cells that synthesize and release pro-insulin and insulin. Therefore, pancreatic duct cells can be a source of pancreatic progenitor cells (Lü et al. 2007). However, it is worthwhile asking whether or not these progenitor cells are MSC. Seeberger et al. showed that pancreatic stem cells could differentiate into osteogenic, chondrogenic, and adipogenic lineages, and also express the transcription factors Pdx-1, Pax-4, and Ngn-3, suggesting that these progenitor cells are MSC that can transform into beta-cells (Seeberger et al. 2006).
Initially, Gershengorn et al. considered that pancreatic MSC could represent an abundant source of islet progenitors, and produce sufficient numbers of ISC for transplants, as fibroblast-like cells residing within the pancreas are multipotent and capable of reversible endoderm-to-mesoderm transitions, just like MSC (Gershengorn et al. 2004). Then the same researchers, as well as Atouf et al., concluded that mouse pancreatic beta-cells do not generate endocrine precursor cells by epithelial–mesenchymal transition (EMT) in vitro (Morton et al. 2007; Atouf et al. 2007).
Using recombinant-based genetic cell tracing, to determine the origin of proliferating fibroblast-like cells in mouse islet cultures in vitro, Chase et al. found that they do not undergo EMT, but represent MSC, like those isolated from BM (Chase et al. 2007).
Experimental approaches, using various phenotypic cell markers (such as nestin, Ngn-3, and c-Met), may improve the isolation of pancreatic progenitor cells. Timper et al. and Eberhardt et al. found that cells expressing nestin can be isolated from human and rodent pancreatic islets, and can be expanded in vitro (Timper et al. 2006; Eberhardt et al. 2006) . Nestin-positive islet cells display endocrine differentiating capacity, so this intra-cytoplasmic filament protein could correspond to a pancreatic stem or progenitor cell marker (Timper et al. 2006; Eberhardt et al. 2006; Chou et al. 2003) . Indeed, insulin, glucagon, Pdx-1/Ipf-1 expression and low-level insulin secretion were detected in cultures of nestin-positive islet-derived stem or progenitor cells, suggesting that such cells can participate in the neogenesis of islet endocrine cells.
Stem or progenitor cells were independently isolated from pancreatic ducts and islets, in both developing and adult mice that, under specific differentiation conditions, were able to release insulin in a glucose-dependent manner (Seaberg et al. 2004). After differentiation, these cells expressed specific developmental pancreatic endocrine genes (such as Ngn-3, Pax-4, Pax-6, and Pdx-1). Some of these differentiated cells were nestin-positive and some were negative; yet, all cells had Ngn-3, a specific marker for pancreatic endocrine growth and development. The important role of the Ngn-3 gene in islet-cell progenitor activation, and in expansion of the beta-cell mass, has been confirmed in a mouse model of injured pancreas (Xu et al. 2008). In that study, the authors showed that activation of Ngn-3 gene expression increased beta-cell hyperplasia, whereas knockdown of Ngn-3 impaired injury-induced beta-cell generation.
Moreover, the report shed light on a new population of Ngn-3-positive progenitor cells purified from adult mouse pancreas—specifically located in the duct lining. These cells were shown to differentiate into functional beta-cells in vitro, and after culture in embryonic pancreas explants. Suzuki et al. isolated pancreatic progenitor cells from neonatal and adult mice, using flow cytometry and clonal analysis, with the HGF receptor c-Met as a phenotypic marker. They determined that the interaction of c-Met and HGF is essential, for growth and differentiation of pancreatic stem or progenitor cells during development, and that it contributes to regeneration and homoeostasis of the pancreas in adults (Suzuki et al. 2004). While monolayer of human islet when expanded in culture in vitro was associated with loss of function and senescence (Beattie et al. 2002) (Table 14.3).
14.12 Umbilical Cord Blood Derived: MSC to Treat Experimental Diabetes
Human UCB-MSC are able to differentiate into functional ISC which, after administration in multiple low doses (50 mg/kg per 2 days) to the liver of STZ-induced diabetic mice, can normalize and stabilize glycemic levels (Chai et al. 2008). Ende et al. also reported success with human-UCB-MSC, as a treatment for IDDM and non-IDDM in mouse models (Ende et al. 2004a, b). Human umbilical cord blood mononuclear-cell injections into the orbital plexus of obese B6.Y Lep(ob) mice, with spontaneous development of non-IDDM, improved blood glucose levels and survival rates, and led to normalization of glomerular hypertrophy and tubular dilatation (Ende et al. 2004a). Furthermore improved glycemic profiles, associated with histological improvement of insulitis, were obtained after intravenous administration of human UCB-MSC to 25 NOD IDDM mice with insulitis (Ende et al. 2004b). They concluded that UCB-MSC may represent a potential source of diabetic cell replacement, because of their availability, their low risk for immune rejection, and increased capacity for expansion.
14.13 Human UCB-MSC-Derived ISC to Treat Experimental Diabetes
Yan-Hua Hu et al. showed that MSC derived from human UCB have good research and application prospects in the treatment of diabetes. They induced UCB-MSC to ISC, by an inducing protocol with extracellular matrix gel. BALB/C nude mice were made hyperglycemic by intraperitoneal injection of streptozotocin. The diabetic mice were transplanted with 1 × 107 ISC under the renal capsule or with phosphate-buffered saline as a control.
After transplantation, the grafts were analyzed by immunocytochemistry for the expression of human insulin; the serum human insulin levels were measured; and blood glucose and body weight status were monitored. They noted that immunofluorescence showed that numerous ISC under the kidney capsule were insulin-positive. On day 14 after transplantation, the serum human insulin level of the treatment group (n = 9) averaged 0.44 ± 0.12 mU/L, which was higher than that of the control group (n = 9) that did not express insulin (t = 10.842, P < 0.05). The diabetic mice remained hyperglycemic, and kept losing body weight after ISC transplantation, and there was no significant difference in the control group. They found that ISC differentiated from UCB-MSC expressed human insulin after being transplanted into streptozotocin (STZ)-induced diabetic mice, but did not find a sustained correction of hyperglycemia and body weight loss.
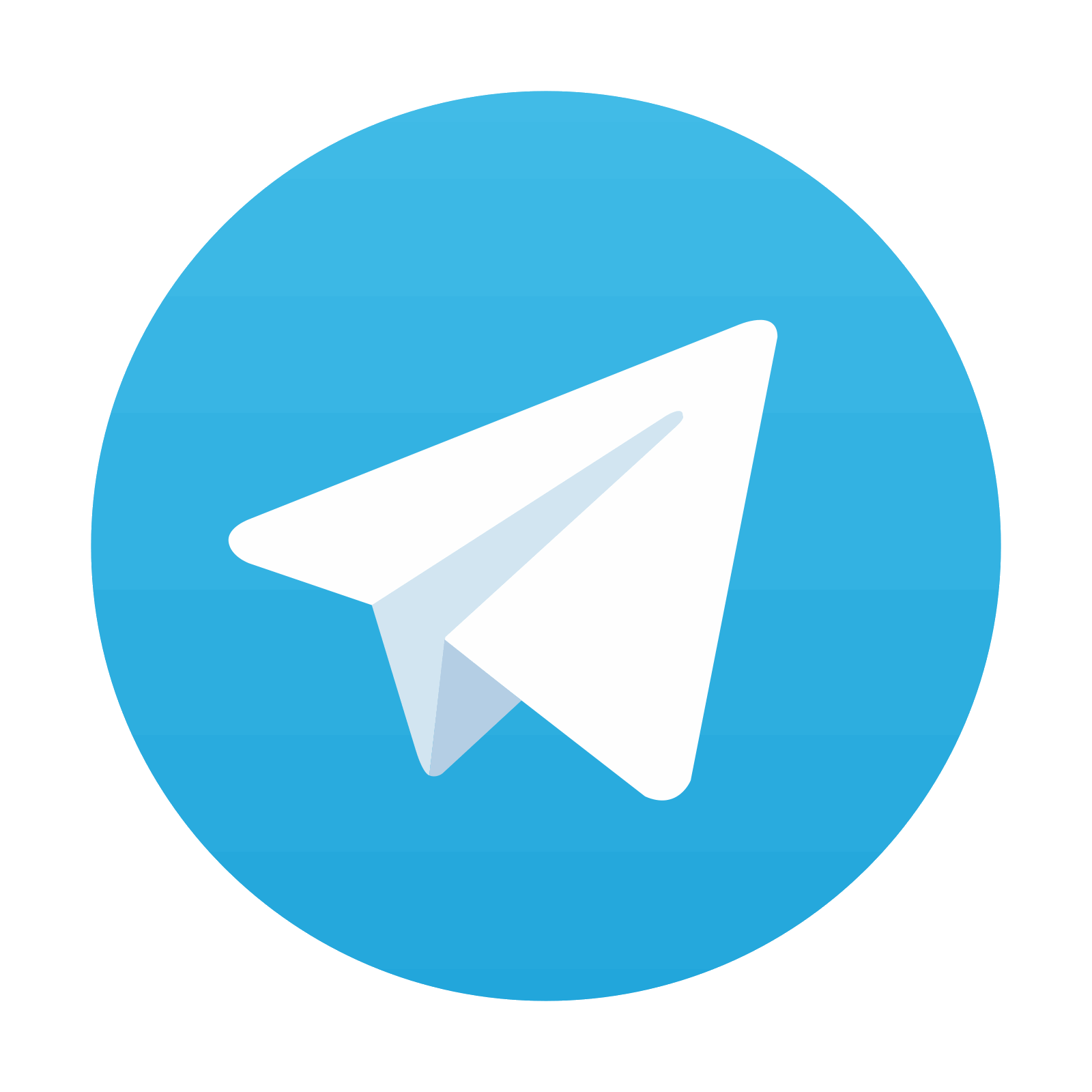
Stay updated, free articles. Join our Telegram channel
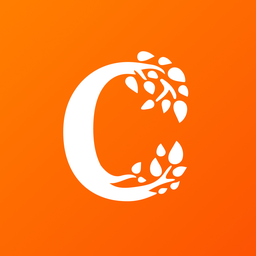
Full access? Get Clinical Tree
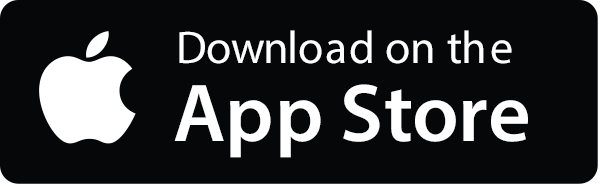
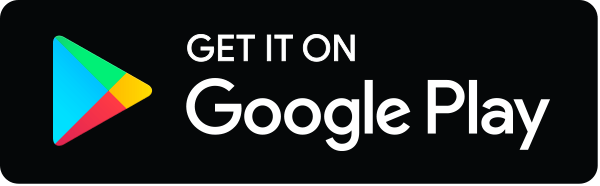