Fig. 13.1
MicroRNA processing and the RISC complex. The gene encoding the microRNA is transcribed to produce a pri-miRNA which is cleaved by DROSHA to produce a pre-miRNA. The pre-miRNA is exported from the nucleus to the cytoplasm via exportin-5. In the cytoplasm, the pre-miRNA is cleaved again by DICER to produce a miRNA duplex. The leading strand then separates from the duplex and associates with the RISC complex at the target gene sequence on mRNA transcripts. The target gene is silenced through cleavage of the mRNA transcript and/or suppression of translation
The miRNA duplex is then incorporated into the RNA-induced silencing complex (RISC) where the two strands are separated and the nonselected passenger strand degraded, resulting in a mature miRNA guide strand which is loaded into the complex [7]. This requires the core component argonaute protein and possibly an additional unidentified helicase [7, 12]. The guide strand in association with the RISC complex binds to the complementary sequence on the target mRNA transcript. This interaction acts to either repress translation or promote cleavage and degradation of the mRNA transcript, effectively resulting in suppression of the targeted gene [7]. See Fig. 13.1 for schematic demonstrating miRNA-mediated gene silencing through incorporation into the RISC complex.
The cellular outcomes of gene silencing by miRNAs vary widely depending on the function of the target gene and physiological context. Initial studies of miRNA investigated the role of these molecules in worm development. A number of miRNAs have been identified in C. elegans which target specific transcription factors expressed only in early stages of development whose downregulation hastens transition to the next step in the developmental program (i.e., lin-4, let-7) [7]. In other physiological processes, the role of miRNAs is often more complex. For example, a miRNA might target a gene encoding a suppressor of a particular signaling pathway. Silencing of this gene by a miRNA will decrease production of the suppressor and lead ultimately to upregulation of the signaling pathway. For example, miR-210 represses COX10, relieving its suppression of the production of reactive oxygen species in the developing placenta [13]. Other miRNAs play vital roles in complex negative feedback loops, holding signals in dynamic balance depending on cellular and environmental context. The role of miR-146 in regulation of NF-κB signaling is a notable example [14, 15]. See Fig. 13.2 for a schematic outlining possible outcomes of deranged miRNA expression.
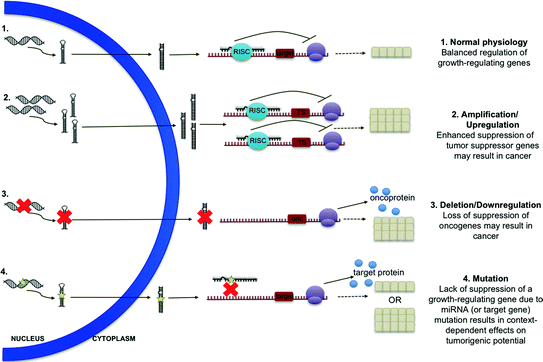
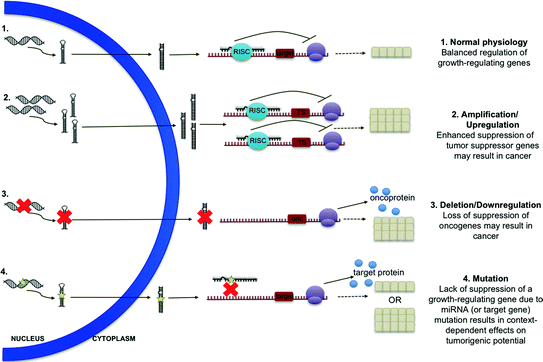
Fig. 13.2
MicroRNA defects contributing to carcinogenesis. In normal physiology, miRNA regulate growth in a balanced fashion. Amplification or upregulation of miRNAs which target tumor suppressor genes and deletion or downregulation of miRNAs which target oncogenes may result in cancer. Mutation of miRNAs or their target sequences may result in context-dependent effects on tumorigenesis
Roles of miRNAs and Associated Proteins in Cancer Biology
As with genes encoding protein products, those encoding miRNAs are also subject to amplification, deletion, mutation, and other derangements which may contribute to human disease (see Fig. 13.2). Indeed, miRNAs and associated proteins have been implicated in both hematologic and solid tumor malignancies. Consistent with increased “genetic activity” in cancer, miRNAs are globally downregulated in the malignant cell [16]. However, particular miRNAs, as well as components of the miRNA processing machinery, have been shown to play varied roles in tumor initiation and disease progression.
A variety of miRNAs have been shown to be upregulated in cancer. Similar in concept to “oncogenes,” such miRNAs are termed “oncomiRs” [14]. These oncomiRs generally exert their oncogenic effect by suppressing genes encoding antiproliferative, proapoptotic, or other antitumor factors. One of the first examples of an oncomiR and the first example of oncomiR addiction was miR-21, a miRNA found to be overexpressed in human tumors [17–20]. Several groups have shown in mouse models that forced overexpression of miR-21 or expression in the presence of an oncogene such as Kras results in malignancy [17, 21]. Furthermore, deleting miR-21 or restoring normal levels of miR-21 expression results in reduced cancer incidence or regression of primary tumors, respectively [17, 22]. In order to exert its oncogenic effects, miR-21 is thought to repress PTEN and other targets to suppress proapoptotic pathways [14, 23]. Another notable example is the miR-17–92 cluster, a group of miRNAs expressed polycistronically, which is amplified in a number of human malignancies [24–27]. Its targets include the proapoptotic gene BCL-2-like protein 11 (BIM) and tumor suppressor PTEN [28].
Many miRNAs have tumor suppressive properties, suppressing genes with oncogenic potential. Similar to tumor suppressor proteins, loss of a tumor suppressive miRNA through deletion or mutation increases susceptibility to malignancy. Such miRNAs are often downregulated or absent in malignant cells. The first miRNAs implicated in such a role were miR-15 and miR-16a, both members of the miR-15–16 cluster [29]. The cluster is located within a fragile site which is frequently deleted in B-cell chronic lymphocytic leukemia [29]. The cluster normally exerts tumor suppressive effects through suppression of cyclin D1, and restoration of miR-15–16 indeed results in decreased cellular proliferation [30–32].
In many cases, miRNAs have context-dependent roles in cancer. As mentioned previously, miR-146 plays a complex role in signaling through NF-κB which may have oncogenic or tumor suppressive effects depending on the interplay of other signaling pathways [14]. Similarly, miR-29 has been shown to have tumor suppressive effects in aggressive CLL by targeting the oncogenes such as TCL1, but recent evidence suggests that miR-29 might also act as an oncomiR by targeting the tumor suppressor peroxidasin [33–35]. More generally, the functions of various miRNAs may be altered through mutation of the miRNA itself or mutations in the sequence of potential target genes, with various effects on tumorigenicity.
Proteins involved in miRNA processing and function have also been implicated in tumorigenesis. A number of perturbations in the miRNA processing machinery have been identified which contribute to human cancers. DICER has been most studied in this context as the central molecule in miRNA processing due to the observed global downregulation of miRNA in cancer [16]. Indeed, DICER is often hemizygously deleted or downregulated in human tumors [36–45]. Interestingly, homozygous deletion does not increase tumorigenicity though miRNA expression is absent, suggesting that DICER itself is a haploinsufficient tumor suppressor [36, 46].
Association of miRNAs with Breast Cancer
In the normal physiology of breast, the expression of various miRNAs directs different stages of mammary gland development, as well as the transition from the mature breast to lactation and involution [47, 48]. Deregulation of physiologic miRNA expression and aberrant suppression or upregulation of various miRNAs may contribute to tumorigenesis, disease progression, and response to therapy. Those miRNAs which have been most widely studied are mentioned here. A more complete, though not exhaustive, list can be found in Tables 13.1 and 13.2.
Table 13.1
Example MicroRNAs with significance in breast cancer
MicroRNA | Significance | Example gene targets | References |
---|---|---|---|
let-7 | Generally downregulated, upregulated in lymph node negative disease, expression associated with luminal subtypes | ERα, IL-6 | |
miR-10b | Downregulated | HOXD1, Tiam1 | |
miR-16 | Upregulation sensitizes HER2+/ER+ cancer to tamoxifen, downregulation associated with tamoxifen resistance | MYB, WIP1 | |
miR-17–92 | Frequently deleted, upregulated in ER-lymph node negative cancer | HIF1, STAT3, AIB1, BRCA1, ERα | |
miR-21 | Upregulated | PTEN, PDCD4, TIMP3, RHOB, BCL2 | |
miR-26 | Upregulated in ER+ disease | EZH2, MTDH, MCL-1 | |
miR-27 | Upregulated in disease progression | FOXO1, ZBTB10, CYP1B1 | |
miR-29 | Upregulated in both ER+ and PR+ disease, reduced expression associated with basal-like subtype | SPARC, TTP, DNMT3b | |
miR-30 | Upregulated in both ER+ and PR+ disease | UBC9, ITGB3, FOXD1, AVEN | |
miR-125 | Downregulated | ERBB2, ERBB3, BAK1, MUC1 | |
miR-126 | Downregulated in cancer and metastatic disease | VEGF-A, PIK3R2, IRS1 | |
miR-145 | Downregulated | MUC1, ERα | |
miR-146 | Context-dependent effects as outlined in text | IRAK1, TRAF6, BRCA1, BRCA2 | |
miR-155 | Upregulated, associated with chemoresistance | FOXO3, SOCS1, RHOA | |
miR-185 | Downregulated in ER+ disease | SIX1 | |
miR-200 | Downregulated in epithelial–mesenchymal transition | ZEB1, ZEB2 | |
miR-205 | Downregulated in cancer and epithelial-mesenchymal transition, expression associated with ductal morphology | ZEB1, VEGF-A, ERBB3 | |
miR-206 | Generally upregulated, downregulated in ER+ | ERα, GATA3, SRC3 | |
miR-210 | Upregulated in cancer and triple negative disease, associated with invasiveness | MNT, RAD52 | |
miR-221 | Generally downregulated, upregulation in invasive disease, associated with tamoxifen resistance | ERα, p27KIP1, TRPS1 | |
miR-326 | Downregulated in late stage disease, associated with chemoresistance | MRP-1 | [161] |
miR-335 | Downregulated in metastatic disease | SOX4, TNC | |
miR-373 | Upregulated in metastatic lesions | CD44, TXNIP, RABEP1 | |
miR-375 | Upregulated in progressive lobular carcinoma, downregulated in tamoxifen resistance | MTDH, RASD1 | |
miR-520 | Upregulated in metastatic lesions | CD44, TGFBR2 |
Table 13.2
Example circulating MicroRNAs associated with breast cancer
MicroRNA | Significance | References |
---|---|---|
let-7 | Serum biomarker for breast cancer | [169] |
miR-10 | Increased serum concentration associated with metastatic disease, specific for bone metastasis | |
miR-19 | Serum biomarker for inflammatory breast cancer, higher levels associated with improved outcome in HER2+ metastatic disease | [172] |
miR-21 | Serum biomarker for breast cancer, serum levels correlated to distant metastasis and lymph node positivity | |
miR-30 | Serum biomarker for breast cancer (decreased levels) | [86] |
miR-34 | Increased serum concentration associated with metastatic disease | [170] |
miR-92 | Serum biomarker for breast cancer (decreased levels), decreased serum levels associated with lymph node positivity | [81] |
miR-122 | Increased serum concentration associated with metastatic recurrence | [173] |
miR-125 | Increased serum levels associated with chemoresistance | [83] |
miR-155 | Higher serum levels in PR+ breast cancer | [174] |
miR-181 | Serum biomarker for breast cancer (decreased levels) | [175] |
miR-182 | Serum biomarker for breast cancer, higher serum levels in PR− breast cancer | [88] |
miR-195 | Serum biomarker for breast cancer, differentiates breast from other malignancies | |
miR-210 | Serum biomarker for breast cancer, increased serum levels associated with lymph node positivity and resistance to traztuzumab | [82] |
miR-214 | Serum biomarker for breast cancer, increased serum levels associated with lymph node positivity | [177] |
miR-373 | Increased serum concentration associated with lymph node positivity | [85] |
OncomiRs
As previously discussed, though miRNAs are generally considered to have suppressive properties, multiple miRNAs have been implicated as oncomiRs in breast cancer, most of which function through the suppression of tumor suppressors. One such oncomiR is miR-155, a miRNA which targets the tumor suppressor SOCS1 directly, thereby indirectly activating JAK-STAT signaling [49]. Similarly, miR-181 directly targets the tumor suppressor ATM and has been shown to support malignant transformation and tumorigenesis in both cell culture and mouse models, respectively [50]. Other potential oncomiRs have been identified as biomarkers. miR-21 was initially identified as a biomarker in breast cancer and subsequently shown to target a number of tumor suppressors to promote breast tumorigenesis and metastasis [51–53]. See Table 13.1 for a partial list of miRNAs identified as oncomiRs in breast cancer.
Tumor Suppressive miRNAs
In line with the suppressive functions of miRNAs, a larger number have been identified as potential tumor suppressors in breast cancer. One of the first miRNAs ever identified and one of the first to be designated a bona fide tumor suppressor in breast cancer was let-7 [54]. Let-7 targets the oncogene RAS and is a regulator of the mammary stem cell population [54, 55]. Upregulation of this miRNA forces stem cells to exit the self-renewing population thought to harbor cancer-initiating cells [55]. Another regulator of the stem cell population is miR-200 which functions in a similar manner [56]. miR-200 also suppresses tumorigenesis by promoting mesenchymal–epithelial transition (MET) and targeting the AKT prosurvival pathway [57–60]. Another identified tumor suppressor is miR-125 which was shown to directly target HER2 in this aggressive subtype of breast carcinoma [61]. See Table 13.1 for a partial list of miRNAs identified as tumor suppressors in breast cancer. See Fig. 13.3 for a list of miRNAs involved in breast carcinogenesis and disease progression.
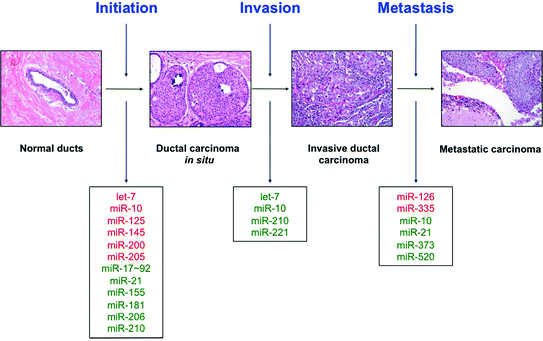
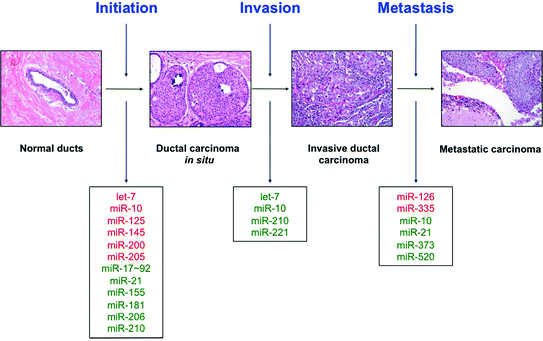
Fig. 13.3
MicroRNAs in tumor progression. Various miRNAs contribute to breast tumor initiation, progression to invasive disease, and metastasis to distant sites. Those miRNAs which are downregulated at each step are marked in red, and those miRNAs which are upregulated at each step are marked in green
Context-Dependent miRNAs
In addition, to the context-dependent miRNAs discussed previously, many miRNAs have been shown to have varied and conflicting roles in breast cancer. A notable example is the miR-17–92 cluster, originally identified as a tumor suppressor in breast cancer by targeting cyclin D1 and IL-8 to inhibit the aggressive features of cancer, including proliferation, motility, and invasion [62, 63]. However, more recent studies have found an oncogenic role for this cluster, suggesting that it may have tumor suppressive or oncogenic effects depending on cellular context [64]. It is likely that many miRNAs will ultimately be determined to have multiple and conflicting roles in breast cancer in a context-dependent manner.
miRNAs Involved in Disease Progression
Many miRNAs with no identified role in breast tumorigenesis have been implicated specifically in disease progression and metastasis. Best studied is miR-10b which, though absent or downregulated in primary tumors, is upregulated in metastatic lesions as it is induced by the epithelial–mesenchymal transition (EMT) transcription factor Twist. Homeobox D10 (HOXD10) is targeted by miR-10b, indirectly inducing RAS homolog C (RHOC) to promote invasion and motility [65]. Other miRNAs such as miR-335 and miR-126 have been identified which are suppressed in metastatic lesions and likely inhibit disease progression by targeting genes involved in invasion and motility [66]. In experimental models, reconstituting expression of these miRNAs can suppress carcinoma cell migration and inhibit metastasis in mice [66]. A partial list of miRNAs involved in disease progression can be found in Table 13.1.
miRNAs Associated with Histologic Phenotypes
Though there is currently little evidence associating particular miRNAs with the various morphologies of breast carcinoma, many miRNAs have been associated more broadly with histopathologic phenotypes. For example, a number of miRNAs have been associated with hormone receptor status, an immensely important clinicopathologic feature in predicting prognosis and guiding treatment. For example, miR-191 is upregulated in estrogen receptor (ER) positive cancer compared to ER negative breast cancer [67, 68]. Other miRNAs have been associated with HER2 status, molecular or intrinsic subtypes (to be discussed further in the next section), and broad morphologic categories (i.e., ductal vs. lobular). Many of these are mentioned in Table 13.1.
miRNA Signatures Associated with Breast Cancer
Advancements in microarray technology have made molecular profiling of large numbers of specimens possible. While the molecular profile of a tumor may hint at particular miRNAs which might play a role in tumor initiation and progression, the greatest strength of miRNA profiling technology is the ability to efficiently analyze a large number of tumors to identify miRNA signatures associated with important clinicopathologic variables. A large number of studies have identified miRNA signatures which may predict the clinical behavior of breast tumors. Those best validated will be discussed here.
The Cancer Genome Atlas Network (TCGA) published their landmark study in 2012 outlining the molecular profile of more than 500 breast tumors and approximately 20 normal breast samples [69]. In addition to whole genome sequencing, the study included gene expression analysis and reported on both mRNA and miRNA expression. Clustering analysis revealed 7 subtypes of tumors within the tested tumor subset correlating to mRNA profiling subsets (particularly those correlating to the basal-like intrinsic subtype as predicted by PAM50), hormone receptor status, and HER2 status [69].
Other groups have used this same patient cohort to identify miRNA signatures with clinical significance. These identified signatures are then applied to data from other existing cohorts to assess applicability more broadly. To date, miRNA and integrated miRNA/mRNA signatures have been validated in multiple cohorts to predict both distant relapse-free and overall survival, invasiveness or risk of transition from ductal carcinoma in situ (DCIS) to invasive carcinoma, aggressiveness in inflammatory breast carcinoma, likelihood of metastasis based on tumor-initiating properties, and possibly others [70–73]. The greatest promise seems to lie with the integrated 9 miRNA/11 mRNA signature developed by Volinia and Croce which demonstrated a higher prognostic value in early stage tumors than the popular Oncotype DX and MammaPrint assays [70].
Clinical Relevance of miRNA Expression Data
Though miRNA expression analysis and miRNA profiling of tumors is used widely in cancer research, these methodologies are not currently used in the standard treatment of breast cancer. Many academic groups and commercial companies are exploring the clinical usefulness of miRNA expression data in the diagnosis and management of breast cancer and other malignancies. Though evaluation of single miRNAs with known diagnostic or prognostic significance may prove clinically useful, miRNA signatures will likely be more widely used in the future.
Diagnosis
Though analysis of particular miRNAs or miRNA signatures in breast tumor samples will be useful in patient management once validated, detection and accurate diagnosis of breast cancer remains an invasive process involving biopsy and/or lumpectomy. Much recent work in diagnostics has focused on noninvasive means of detection in order to detect breast cancers early and avoid unnecessary invasive procedures. The miRNAs expressed in normal and malignant tissues are frequently detectable in the body fluids of patients. As miRNAs are remarkably stable in the serum and detection methodologies advancing, it is possible that a simple blood test could detect cancer-associated miRNAs with high sensitivity and specificity [74–76]. In particular, miR-21 has been used in several studies to differentiate patients with and without breast cancer, suggesting this may be a promising candidate serum biomarker [77–81]. A variety of other miRNAs have been identified in the serum whose expression levels correlate to various clinicopathologic features [82–88].
Prognosis
Great advances in molecular pathology have revolutionized breast cancer medicine, identifying particular biomarkers with prognostic significance such as ER/PR and HER2. However, the management dilemma remains that prediction of relapse-free and overall survival is not perfect, and many patients with low risk cancer based on ER and PR positivity, for example, will experience local or distant relapse at various timepoints after the initial diagnosis. New diagnostic molecular assays such as Oncotype DX and Mammaprint attempt to bridge that gap with some success. miRNA and integrated miRNA/mRNA analysis to identify certain prognostic signatures as discussed previously show great promise as alternative or additional tests to determine which patients are most likely to relapse and require more aggressive treatment. Such tests may become commercially available in the near future.
Treatment Response
Resistance to standard chemotherapy is currently one of the major areas of focus in breast cancer research. Though certain markers are known to associate with sensitivity to particular therapies, such as HER2 for Herceptin or ER for tamoxifen, we do not currently have markers of resistance in widespread clinical use. A number of large studies have identified genetic signatures associated with treatment response, including a study that identified miRNA signatures associated with sensitivity to several commonly used chemotherapeutics [89]. A variety of other studies have used miRNA profiling to identify particular miRNAs which are up- and downregulated in drug-sensitive versus drug-resistant cell lines [90–95]. Many of these are mentioned in Table 1. In the study of treatment resistance, miRNAs have become attractive targets, as more therapeutic options exist for overcoming resistance associated with these genetic elements [96]. Current studies have not used this information in clinical decision-making, and further characterization of identified miRNAs and miRNA signatures will be required to apply this data in patient studies.
miRNA Therapeutics
As investigation continues to develop new therapeutics for resistant and difficult-to-treat cancer (i.e., triple negative), miRNAs have recently become attractive targets for therapy. These molecules are minimally antigenic compared to protein and carbohydrate biological drugs and small enough to penetrate a cell as a nanoparticle, liposome, or exosome without the need for potentially dangerous viral vectors [47]. However, tumor-specific drug delivery is a major concern, as systemic exposure to a miRNA or antagomiR (antisense oligonucleotide specific to a particular miRNA) could have unforeseen consequences in other organ systems. Tumor-specific antibody- and ligand-mediated systems will likely help to solve this major challenge [47].
Though miRNA therapeutics have not yet been studied in human patients, a number of potential therapies have been successfully tested in animal models. AntagomiR-10b has been used by Weinberg’s group to inhibit motility and invasiveness of a mouse mammary tumor cell line in a mouse model without adverse effect [97]. AntagomiR-21 has also been used to inhibit angiogenesis and induce apoptosis in a murine breast cancer model [98]. Other groups have used antagomiRs to treat neuroblastoma and cardiac hypertrophy, among others [99–101]. Though only one study has explored miRNA replacement therapy in breast cancer cells in vitro for radio-sensitization, miRNA replacement therapy has been tested with some success in cancers of the liver, colon, and lung using adenoviral, nanoparticle, or lipid-based delivery systems [102–106]. Unfortunately, tumor-specific delivery was not possible in these studies. However, successful treatment without major adverse effects is promising for the development of safe, effective new miRNA-based therapeutics in the near future.
Laboratory Methods
Knowledge of the techniques through which miRNA are purified and analyzed is vital for the accurate interpretation of miRNA data gathered from clinical specimens.
Detection and Quantitation
The analysis of miRNA expression requires isolation of RNA from clinical specimens, including plasma samples, fine needle aspirates, and formalin-fixed tissues. There is a remarkable stability of small RNAs in the blood, particularly in the exosomal fraction, and miRNA can be isolated from the plasma with relative ease [107]. Most commonly, RNA is isolated using commercial kits and reagents, though the underlying methodology is the same. In brief, organic components are isolated through fluid phase separation by centrifugation. Chloroform and phenol in an aqueous buffer are added to the sample prior to centrifugation. A denaturing reagent, often guanidinium isothiocyanate, is also added to denature ribonucleoproteins which may complex with RNA, as well as RNAases. Following centrifugation, nucleic acids (DNA and RNA) will be found in the aqueous phase, lipids in the chloroform interphase, and other organic molecules in the phenol organic phase. The aqueous phase is then mixed with ethanol or isopropanol to precipitate nucleic acids. This mixture can then be applied to a silica-based column (i.e., RNeasy) to isolate total RNA (see Fig. 13.4) [74].
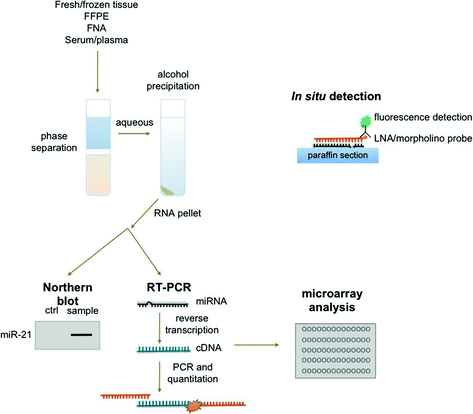
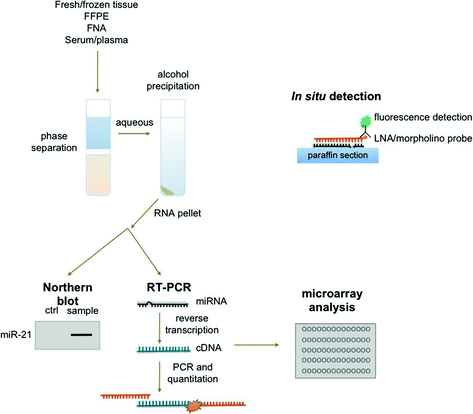
Fig. 13.4
Laboratory methods for microRNA detection in clinical samples. Total RNA may be isolated from tissues and body fluids through phase separation and precipitation in alcohol. Individual miRNAs may be detected using Northern blot or RT-PCR. Microarray analysis can be used to profile a large of miRNAs from a single sample. In paraffin-embedded tissues, individual miRNAs may be detected using fluorescent in situ hybridization and visualized through fluorescent microscopy
Though isolation of RNA from plasma is relatively rapid and simple, miRNA analysis of a tumor often requires fine needle aspirates of the lesion or formalin-fixed paraffin-embedded (FFPE) tissue blocks, as fresh tissue is not frequently available in the clinical setting. Fortunately, the small size of miRNAs is relatively protective against the damaging effects of formalin. Tissue is isolated from paraffin tissue curls treated with xylene by centrifugation. The resulting tissue pellet is treated with the broad specificity enzyme proteinase K to degrade contaminating proteins in the preparation. Nucleic acids are then precipitated in ethanol and total RNA isolated as previously discussed, usually using a commercially available silica-based column (see Fig. 13.4) [74].
As these techniques separate total RNA from the tissue specimen, miRNAs of interest must then be specifically detected. This is normally accomplished in one of two ways: (1) Northern blot or (2) real-time reverse transcriptase polymerase chain reaction (RT-PCR). In Northern blotting, total RNA extracts are separated by charge and size in an agarose or polyacrylamide gel, transferred to a nylon membrane, hybridized to sequence-specific probes, and detected radiographically or by chemiluminescence. While blotting is semiquantitative and best for determining qualitatively whether a miRNA is expressed, RT-PCR is truly quantitative. First, extracted RNA is reverse transcribed by the enzyme reverse transcriptase in a thermal cycler to produced complementary DNA (cDNA). Next, traditional PCR is used to amplify the target miRNA sequence using specific primers. A variety of fluorescent dyes and sequence-specific oligonucleotide probes are commercially available for detection of amplified miRNA sequences which utilize straight fluorescence or Förster resonance energy transfer (FRET), respectively. Direct detection of miRNAs in situ is also possible using locked nucleic acid (LNA) or Morpholino oligonucleotide probes (see Fig. 13.4) [74, 108].
Microarray Analysis
Though the ability to detect specific miRNAs has allowed great advances in our understanding of these molecules in human disease, microarray analysis has allowed the simultaneous investigation of hundreds of miRNAs. Briefly, extracted RNA from a clinical sample is applied to a chip containing hundreds of specific oligonucleotide probes to which miRNAs in the sample will hybridize, allowing detection and quantitation of many miRNAs at once in a single sample. By utilizing probes for housekeeping genes in the microarray, the resulting data can be normalized, allowing comparison of multiple clinical samples. For example, it is possible to determine which miRNAs are up- or downregulated in tumor samples compared to normal tissue or identify miRNA signatures associated with a particular tumor subtype, hinting at potential biomarkers and pharmacological targets (see Fig. 13.4) [74].
Key Points
MicroRNAs are small, noncoding RNAs measuring ~22 nucleotides in length which are processed by the ribonucleases Drosha and DICER from longer transcripts containing a hairpin structure
Mature microRNAs silence genes containing complementary sequences through the RISC silencing complex
A single microRNA may silence multiple genes, and a single gene may be silenced by multiple microRNAs
OncomiRs are microRNAs with oncogenic properties, often through silencing of a tumor suppressor gene
Tumor suppressive microRNAs generally exert suppressive effects by silencing an oncogene
MicroRNAs have a number of context-dependent roles in tumor initiation, disease progression, prognosis, and response to therapy
MicroRNAs are readily detectable in the blood and other body fluids, FNA material, and FFPE blocks
Microarray technology has made possible the detection of hundreds of microRNAs at once in large numbers of tumors, allowing for the development of microRNA signatures associated with various clinical parameters
Though not currently in clinical use, detection of particular microRNAs and microRNA profiling of blood and tumor samples may soon contribute to clinical decision-making as an adjunct to traditional pathologic evaluation
AntagomiRs (antisense oligonucleotides) and miRNA replacement therapy have been used successfully in animal models and are potential candidates for new therapeutics in breast cancerStay updated, free articles. Join our Telegram channel
Full access? Get Clinical Tree