Fig. 16.1
Endogenous neural stem cells and stroke. (a) Stroke invokes a neurobiological response in the affected tissue that is diverse by involving different types of cells, such as astrocytes (glial fibrillary acid protein-positive cells) and microglia (CD11b+ cells), even within 1 h post-stroke. These cells delineate the affected area after occlusion of the middle cerebral artery (MCA) that supply blood to the striatum and medial portion of the neocortex. However, the extent of damage, as well as the neurobiological response evolves over time. Eventually severely affected areas will loose all cells and supportive extracellular matrix to produce a tissue cavity. The peri-infarct area surrounding the cavity is a heterogeneous environment sub-divided by different responses to the ischemic insult. In some areas, astrocytosis is predominant, whereas in others there is total loss of neurons (NeuN+ cells) and in others there is some angiogenesis. Commonly astrocytosis caused by the insult extents through large parts of the remaining striatum and reaches to the lateral ventricles (LV) indicating that these neurobiological changes also affect the site of endogenous neurogenesis, the sub-ependymal zone (SEZ). (b) As the name implies, the SEZ is situated next to the ependymal cell layer that separates the “brain” from the cerebrospinal fluid (CSF) in the lateral ventricles. In the case of stroke, where astrocytosis, or in severe cases even the damage itself, extents to the LV, the activity of the SEZ which represents the endogenous neural stem cell niche is altered. Specifically, neurogenic activity is shifted dorsally and results in a dramatic increase in neural progenitors being present in the ventricular horn, where these migrate along the corpus callosum or blood vessels to invade the damaged striatum (Smith et al. 2012)
The SEZ stretches along the ependymal cell layer that separates brain tissue from the lateral ventricles (LV), where the choroid plexus is producing cerebrospinal fluid (CSF) (Kazanis 2009). Occlusion of the MCA affects the SEZ by tissue astrocytosis extending into the SEZ, as well as actually tissue loss extending to the ventricles in the more extreme cases (Fig. 16.1b). In either case, it is not uncommon that there are structural changes occurring in and around the SEZ that affect its normal physiological role, notably the continuous production of olfactory neurons (Danilov et al. 2012). In brain development, neurons are generated in the centre of the brain, around the lateral ventricles in a region known as the Sub-Ventricular Zone (SVZ), that gradually evolves into the SEZ. During brain development, neurons born in the SVZ migrate outwards along radial glia to form anatomically distinct brain regions. As this process completes, the SEZ nevertheless, retains its ability to generate neuroblasts (A cells) from transit amplifying cells (C cells). Transit amplifying cells are a progenitor type of cell that is derived from neural stem cells (B cells). Together with capillaries, these cells are forming the neural stem cell niche, a specialized form of a neurovascular unit (NVU). In the normal brain, these neuroblasts will migrate along the rostral migratory stream (RMS) to the olfactory bulb, where they will replace olfactory neurons (Kazanis 2009).
However, a disturbance to the SEZ in stroke leads to a shift in its activity from the ventral to the dorsal region, where the ventricular horn is dramatically increasing the number of new neurons being formed (Smith et al. 2012). A variety of strategies have been pursued to increase this endogenous neurogenesis. Especially the use of secreted factors, such as betacellulin, have been very efficient in increasing the proliferation of endogenous NSCs. After a stroke, a significant amount of neuroblasts from the SEZ are diverted away from the RMS towards the damaged striatum where they migrate alongside blood vessels using stromal cell-derived factor-1 (SDF-1) (Cui et al. 2013). There is evidence that these neuroblast-derived cells express DARPP-32, potentially suggesting that these cells replace lost GABAergic output neurons in the striatum and herewith lay the foundation for some of the observed spontaneous recovery. Nevertheless, DARPP-32 is also known to be expressed on olfactory progenitor cells (Perez and Lewis 1992). It remains hence unclear if endogenous neurogenesis after stroke indeed produces striatal neurons or if this population reflects olfactory neurons that have gone astray. Importantly, very few of these newly formed neurons survive in the peri-infarct area, hence questioning their capability to truly replace lost neurons (Thored et al. 2006). Even if these endogenous neuroblasts do not replace lost neurons, they can still significantly affect the fate of the peri-infarct tissue.
Neural stem cells and neuroblasts are known to secrete a variety of growth factors that can rescue damaged neurons (e.g. brain-derived neurotrophic factor, BDNF), but also can influence endothelial cells along which these are migrating. Indeed, certain peri-infarct areas are showing a robust increase in small arteries (Beck et al. 2000). It remains unclear how these vessels are formed, if they are the product of a sprouting angiogenic stimulus or the result of intussusception. Vasculogenesis, a third process of generating new blood vessels, is thought to only occur during development. A neovascular response of peri-infarct tissue is a physiological response to a lack of sufficient nutrient being available to support tissue needs. As a tissue is undergoing hypoxia, hypoxia-induced factor-1 α (HIF-1 α) is upregulated (Alfieri et al. 2011). This in turn engenders a molecular cascade that involves neuronal, as well as vascular elements to dedifferentiate endothelial cells in the vascular bed and promote their proliferation, as well as sprouting outgrowth. A process that has been extensively studied in brain tumors and this sprouting angiogenesis is dependent on high levels of vascular endothelial growth factor-A (VEGF-A) (Ribatti and Crivellato 2012). Intussusception (i.e. parting of a blood vessel), in contrast, is less dependent on VEGF-A, but is induced by peri-vascular cells protruding through the lumen, while depositing extracellular matrix to create a gap in between two vessels. In both cases, signalling of neuroblasts from the SEZ can influence neovascularization (Thored et al. 2007). However, at present there is no evidence that peri-infarct areas with neovascularization are preferentially invaded by endogenous neuroblasts. Moreover, it remains unclear if neurogenesis is indeed causally linked to improvements of behaviour (Thored et al. 2006) or if it is merely an epiphenomenon.
Transplantation of Neural Stem Cells
Spontaneous recovery and the improvements observed with neurorehabilitation, nevertheless, are limited and most patients remain severely impaired. Although increasing endogenous neural stem cells production and integration could provide greater improvements in the future, it is important to also consider if exogenous supplementation of new cells in the peri-infarct areas could exceed the outcome observed after spontaneous improvements.
Over the past two decades, developing brain tissue has been a source of interest for transplantation. Due to the specific timing of neurogenesis and gliogenesis in the developing brain, it is possible to harvest brain tissue from fetuses that is predisposed to develop into neurons or astrocytes. Importantly, neurogenic tissue will also produce astrocytes, but material collected later in development has a lower propensity to produce neurons (Rosser et al. 2003). Tissue from earlier developmental periods harbors the risk of tumor formation due to the cells being pluri-potent (i.e. can produce multiple organs) rather than multi-potent (i.e. multiple cells from the same organ). Therefore derivation of the primary material will be essential to characterize its neurobiological properties and how these lead to the survival and integration of the materials, as well as behavioral improvements.
Early studies using fetal tissue pieces containing a heterogeneous mix of partially differentiated neurons/astrocytes, neural stem cells (NSCs), progenitors, endothelial cells (ECs), as well as microglia. Little attention was also given to the specific developing brain regions from which these were derived. From the rat, tissue was typically derived between E14-E16 that is considered the neurogenic period, which corresponds to week 8–10 during human development. Initial experiments were encouraging with tissue pieces surviving and demonstrating some connectivity between host and graft in terms of vasculature, as well as axonal projections. Injections into the lesion cavity resulted in some improvements of behavioral functions, but this was influenced by the time of transplantation, as well as the age of the donor (Grabowski et al. 1994). It was generally thought that these improvements were due to functional integration of graft tissue. However, there was evidence that if immunosuppression was not optimal there was a risk of an immune rejection. Two key elements in fetal tissue grafts were thought to induce a host immune response: (1) Endothelial cells from the graft established functional connections with the host vasculature and hence exposed foreign Major Histocompatibility Complex (MHC) molecules to immune cells travelling in the circulating blood (akin to solid organ grafts) and (2) Microglia contained within fetal tissue were highly immunogenic and could even mount a graft-versus-host response. A further consideration in fetal tissue grafts was the poor survival that was thought to be due to partially differentiated cells undergoing apoptosis after implantation (Emgard et al. 2003). Neural stem cells within these tissue pieces were hence thought to potentially produce differentiated cells upon implantation without incurring a major risk of an immune response.
Isolation of neural stem cells and the generation of cells lines was hence first achieved in rodent cells (Marone et al. 1995), but rapidly followed by the generation of human neural stem cell lines (Villa et al. 2000). Although it is possible to derive primary human neural stem cells for implantation, their in vitro propagation is limited, as with an increasing number of passaging the cells gradually shift phenotype which can lead to senescence (so called Hayflick phenomenon) or changes in their differentiation profile. Controlling their proliferation and differentiation is therefore advantageous to develop NSCs into a large scale population of cells which could be used to treat a large number of patients, as would be required for stroke. One way to achieve this is to conditionally immortalize cells. Immortalization of cells leads to their continued proliferation, but does not provide a reliable control over their differentiation. In contrast, conditional immortalization defines the conditions under which cells proliferate and differentiate, hence providing control over cell fate and improving the safety of the cell line. Moreover, this conditional immortalization provides greater stability to the cell lines in terms of maintenance of specific phenotypes and hence allows definition of quality control procedures.
A further important change in the application of both primary cells and cell lines in stroke is that these are no longer implanted as a piece of tissue, but individual cells are suspended in a vehicle that serves to inject cells. Injection of suspension grafts by themselves into the lesion cavity, however, does not lead to the formation of a tissue. Instead injected cells migrate into host tissue and are therefore preferentially injected into the peri-infarct tissue to facilitate their integration in host tissue. Although a variety of peri-infarct injection sites have been used to successfully improve outcome with neural stem cells, intracerebroventricular injection of cells leads to poor survival and no improvement in outcome (Smith et al. 2012). Importantly, human neural stem cells show very little migration away from the injection site (Smith et al. 2012), whereas mouse neural stem cells even migrate from one hemisphere to another to improve outcome (Modo et al. 2002). Although these experiments provide evidence that certain sites will not be conducive to improve behavioral outcome, the diversity of injection sites peri-infarct with recovery suggest that the specific peri-infarct location might not be as important. It currently remains unclear if a single injection could be sufficient to improve outcome irrespective of peri-infarct location or if multiple injections covering the peri-infarct region would still improve outcome.
The topology of the lesion further carries significance in the observed deficits, as well as the observed recovery after peri-infarct injections (Smith et al. 2012). Occlusion of the middle cerebral artery affects a vast territory of brain tissue. Functional deficits and the resulting behavioral impairments are hence a consequence of which anatomical regions are affected by the stroke (Crum et al. 2013). Consequently, implantation of cells peri-infarct is likely to affect functions that are subserved by these peri-infarct regions or those that are connected. The topological reach of the stroke therefore is an important modulator of the efficacy of neural stem cell efficacy (Smith et al. 2012). Although initial studies considered recovery to be mediated through neuronal replacement, increasing evidence suggest that the more important mechanisms are astrocytic differentiation that can lead to a scavenging of excitotoxic glutamate, modulation of microglia activity and the resulting inflammatory response, as well trophic factor support to dying neurons. Interestingly, an interaction of neural stem cells with host vasculature can potentially dramatically influence these mechanisms. For instance, blocking of the effects of vascular endothelial growth factor from human neural stem cells significantly affects behavioral recovery (Horie et al. 2011). Nevertheless, establishing mechanisms of recovery is extremely challenging as multiple signalling interactions occur and affect different physiological processes (Drago et al. 2013). Interfering with one of these can affect the others and hence complicate the investigation of their specific involvement in recovery.
Nevertheless, translation of cell therapy for stroke is not dependent on the identification of a mechanisms of recovery (Chopp et al. 2009). Although mechanism of recovery are essential to ensure optimal efficacy and potentially improving efficacy, clinical translation does not require a detailed understanding of how a therapy works, but is contingent on proven efficacy and safety. To date, three clinical trials have been conducted for intracerebral cell injections in stroke. The first clinical translation applied a human teratocarcinoma cell line that produced post-mitotic cholinergic neurons upon treatment with retinoic acid. Preclinical studies indicated a dose-dependent efficacy of these cells in a rodent model of stroke and there was no evidence of ill-effects (Borlongan et al. 1998). A phase I clinical trial showed no ill-effects of the cells or the stereotactic surgery (Kondziolka et al. 2000), but a phase II clinical trial only revealed minor improvements (Stilley et al. 2004) with post-mitotic cell survival beyond 27 months and a relationship between peri-infarct glucose uptake and motor performance. No further trials were conducted using this cell line. Due to the shortage, logistical difficulties, as well as the ethical concerns regarding the use human fetal tissue material (Rosser et al. 2003), porcine-derived tissue was considered as an alternative source for transplant material. However, a clinical trial using porcine CNS fetal tissue was abandoned after two patients developed side-effects (worsening motor deficits and seizures) (Savitz et al. 2005). More recently, the first clinical trial using a human neural stem cell line that was conditionally immortalized using the c-myc under tamoxifan control completed a phase I clinical trial without any ill-effects being apparent after 12 months post-injection (Sinden et al. 2012). Extensive preclinical testing preceded this translation (Smith et al. 2012), as well as the development of a large cell bank that can be used to treat a significant number of patients using the same “cell product”, facilitating quality control and ensuring that each patient receives the same treatment. Still, phase II trials will need to establish if indeed this approach can successfully improve outcome. The preclinical development, as well as the clinical translation, provides a framework for future approaches.
Tissue Engineering Using Neural Stem Cells and Biomaterials
Even if peri-infarct neural stem cell implantation and/or endogenous neurogenesis will result in clinically relevant efficacy, these approaches do not replace the lost tissue and hence a large tissue cavity remains. To allow neural stem cells to be retained within the cavity requires the use of biomaterials that provide a structural support (Bible et al. 2009b). Bioscaffolding has been extensively used in other organ tissues and even in the spinal cord. Nevertheless, there is a general lack of studies in the brain (Orive et al. 2009). Tissue engineering in the brain faces two major technical hurdles (Bible et al. 2009a): (1) Delivery of the material needs access through the skull. This can either be using a minimal approach of delivery through a drill hole or by means of a craniectomy. A craniectomy is very invasive, changes intracranial pressure and is a potential source for opportunistic CNS infections. Injections through a Burr hole in the skull are hence favorable, but require the material to fit through a thin needle. (2) Delivery of a scaffold requires knowledge regarding the location and volume of tissue to be replaced. Injection of a large volume of material inside existing brain tissue will lead to a dramatic increase in pressure and cause tissue damage. It is therefore essential to use non-invasive imaging, such as Magnetic Resonance Imaging (MRI), to provide surgical planning and guide the injection of the material for in situ tissue engineering (Bible et al. 2009a).
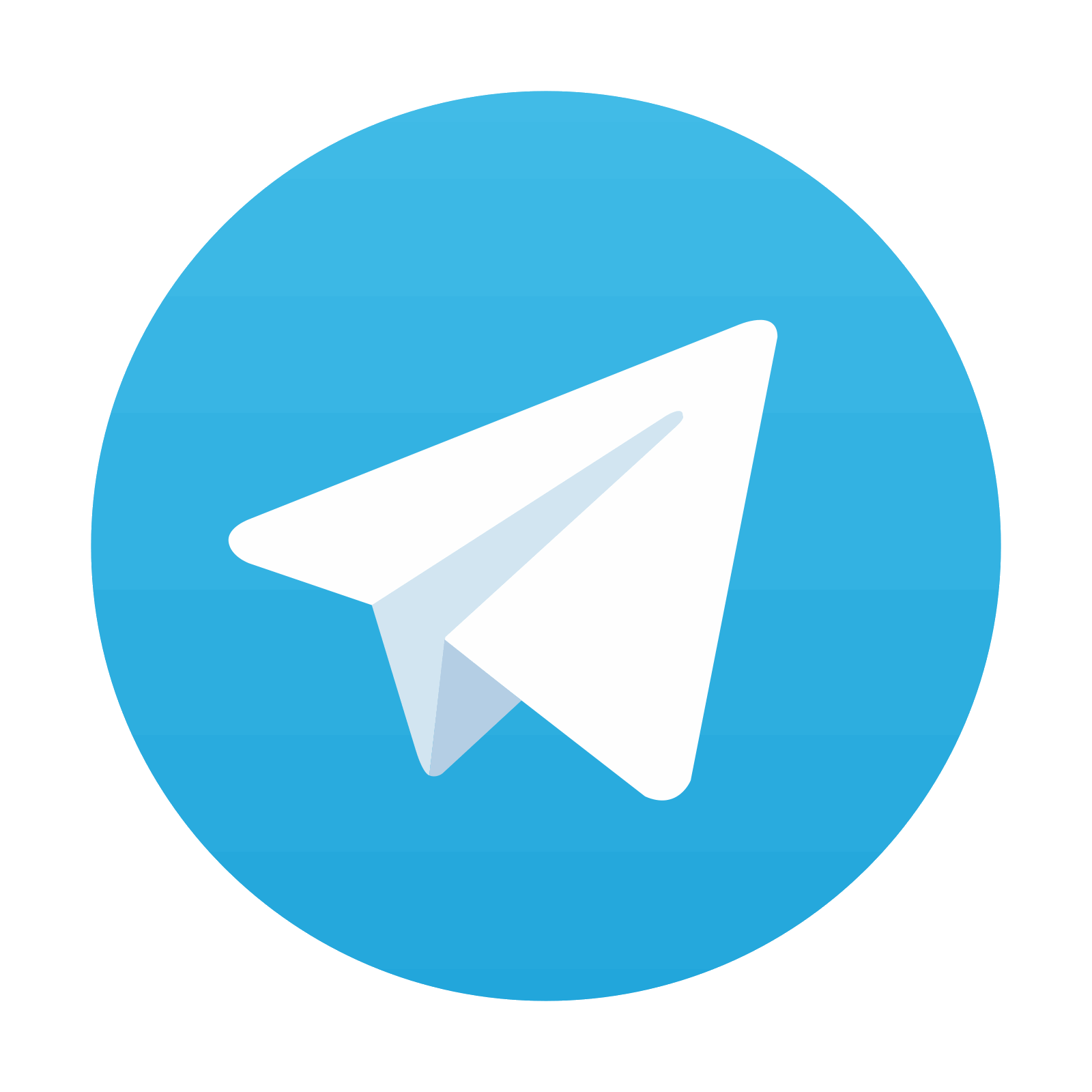
Stay updated, free articles. Join our Telegram channel
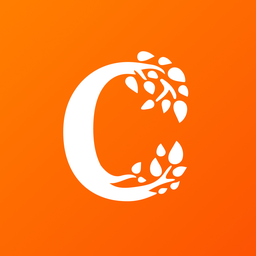
Full access? Get Clinical Tree
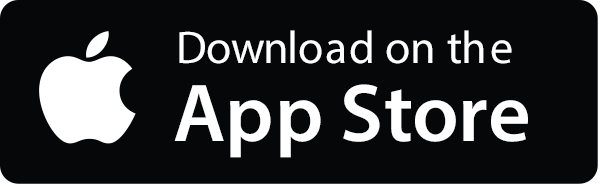
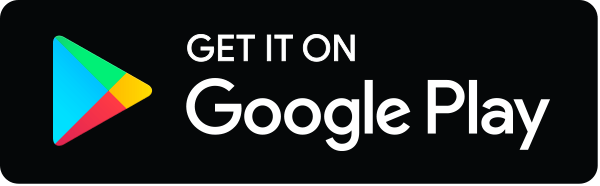