and Winfried G. Rossmanith2
(1)
Lenzkirch, Germany
(2)
Ettlingen, Germany
11.1 Feedback Control
11.2 Regulator Circuits
11.2.1 Pressure and Stress
11.2.2 Calcium Metabolism
11.3.1 Regulation of GnRH
11.3.3 Puberty
11.3.4 Menopause
11.4 Glucose Metabolism
11.4.1 The Origin of Blood Glucose
11.4.5 Insulin-Dependent Procedures
11.5 Appetite and Hunger
11.5.1 Central Nervous System
11.5.4 Enteric Nervous System
11.5.7 Mechanoreceptors
11.6 Growth
11.6.1 Epiphyseal Cartilages
11.6.3 Regulation by Hormones
11.7.4 Regulation of Ecdysis
11.8.5 The Role of Oxytocin
In this chapter we will present examples of endocrine regulation. A single hormone in the endocrine system is something like an individual in a family tree. Different sources exist which determine the creation and development of a single person, and his or her progeny are dependent not on him or her alone, but also on many other people.
That is like the release of a hormone. From everywhere, messengers touch the receptor of the hormone-producing cell, some force release, others retention, some new synthesis, others arrest. An endocrine cell has to integrate all these different influences and decide what to do. This cannot be achieved by intelligence, but by an interplay of control elements in the related signal transduction pathways of the different receptors or by complex interaction while activating genes, or both.
If a cell actually releases an hormone, the stimulating forces prevailed. These are, however, often blocked by high concentrations of the very same hormone, or by other, downstream hormones. For example, gonadotropin-releasing hormone (GnRH) triggers in the pituitary release of luteinizing hormone (LH) and follicle-stimulating hormone (FSH); these trigger in the gonads estradiol and testosterone synthesis. The latter are, in turn, strong inhibitors of GnRH release and LH/FSH secretion.
11.1 Feedback Control
Figure 11.1 demonstrates a simple feedback control pathway. In the thyroid, on stimulation by thyroid-stimulating hormone (TSH ), thyroxine (T4) is synthesized and released (1 in Fig. 11.1). By T4 and by triiodothyronine (T3) derived therefrom through the action of deiodinase, however, TSH release in the pituitary is blocked (2 in Fig. 11.1). TSH release is stimulated by thyrotropin-releasing hormone (TRH ; 3 in Fig. 11.1). This TRH release (4 in Fig. 11.1) is equally blocked by T4 (5 in Fig. 11.1) , whereby T4 has to cross the blood–brain barrier .
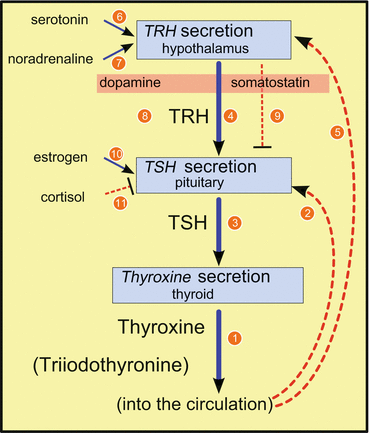
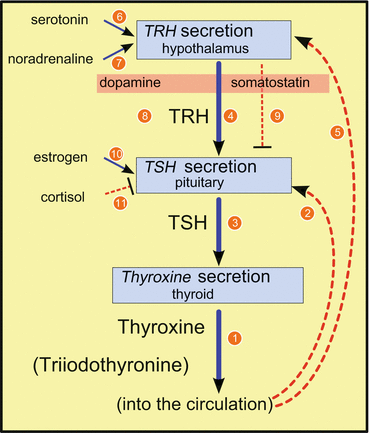
Fig. 11.1
Synthesis of thyroxine and its control. Blue, continuous lines indicate stimulation, red, broken lines indicate inhibition; numbers are explained in the text. TRH thyrotropin-releasing hormone, TSH thyroid-stimulating hormone
T4 synthesis is additionally controlled, for example, by circadian rhythms not shown here. TRH release in the brain is multiply influenced by other neurons and hormones: by serotonin (6 in Fig. 11.1) and by noradrenaline (7 in Fig. 11.1) . TSH release is negatively influenced by (hypothalamic) dopamine (8 in Fig. 11.1) and somatostatin (9 in Fig. 11.1) . Estrogens in the circulation enhance TSH release (10 in Fig. 11.1), and the glucocorticoid cortisol suppresses TSH formation (11 in Fig. 11.1) .
In very similar ways, other hormones are regulated by different additional hormones, neuronal interactions, and other soluble messengers. In the following pages, we will provide several examples.
11.2 Regulator Circuits
11.2.1 Pressure and Stress
Stress was defined by Selye (1936, 1950) as “the nonspecific response of the body to any demand upon it.” Hunger, injury, coldness, fear, cardiac arrest, exhaustion, capture, and arrest as well as social stress result, according to Selye, in the same unspecific reactions of the organism manifesting themselves in a strong elevation of the levels of glucocorticoids and adrenaline in the circulation. There might be specific reactions to any to these threats, called stressors; however, according to Selye, the unspecific aspect of the reaction should be called stress.
An easily comprehensible experiment with accountants was reported by Lennart Levi and colleagues (for an overview, see Levi 1989). The mode of payoff for the pay slip was changed in a daily manner. On odd days the payoff was due to the number of entries accomplished on that day; on even days there was no such check. Whereas on even days the workload remained as before, the quota increased by 14 % on odd days compared with even days. In parallel, adrenaline and noradrenaline were analyzed in urine. The amount of secreted catecholamines on odd days was 40 % above that on even days. Additionally 11 of 12 women reported fatigue, backache, and pain in the shoulders and arms—only on odd days. They felt hurried.
These women stressed themselves. They did not want to lose income, were especially motivated, but were overshooting and their organisms reacted to this psychic pressure. By psychic strain, obviously, stress can be induced. Stress can thus be regarded as a reaction of the central nervous system manifested in other organs.
Modern endocrinology at the dusk of the twentieth century took the physiology of stress as the interaction of two pillars: the hypothalamic–pituitary–adrenal axis, on the one hand, and the sympathetic nervous system, on the other. Both are coupled by mutual communications between centers in the paraventricular nucleus and the locus coeruleus.
In the paraventricular nucleus, parvocellular neurons synthesize corticotropin-releasing hormone (CRH), arginine vasopressin (AVP), or CRH and AVP. After CRH release into the median eminence portal system, ACTH and endorphin s are released in the pituitary. This ACTH stimulates in the adrenal glands adrenaline production and release (see Sect. 7.1) and steroid hormone synthesis, above all synthesis of glucocorticoids.
In the locus coeruleus, noradrenergic neuron s particularly stimulate sympathetic nerve cells . The locus coeruleus nerves project, for example, to the parvocellular neurons of the paraventricular nucleus, and these latter project to the noradrenergic neurons of the locus coeruleus in a mutual way; in this interaction CRH and AVP act as neurotransmitters. The entire system is further controlled by unknown zeitgebers —in any case CRH , AVP, and ACTH are released in one to three pulses per hour and in a daily rhythm where evening/night pulses are greater than those during the day (Fig. 11.2). Furthermore, many other neurons from brain areas and peripheral organs act via sympathetic and other nerves on the paraventricular nucleus and locus coeruleus neurons. The limbic system is tightly coupled to the stress response. Other hormones such as neuropeptide Y (NPY) and substance P are also involved: NPY stimulates CRH release and inhibits locus coeruleus neurons, whereas substance P acts in the opposite way (Strakis and Chrousos 1997).
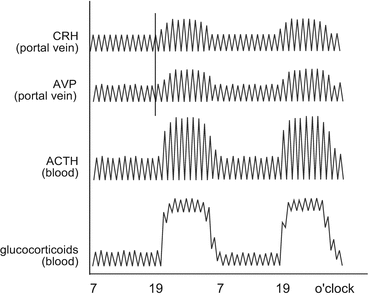
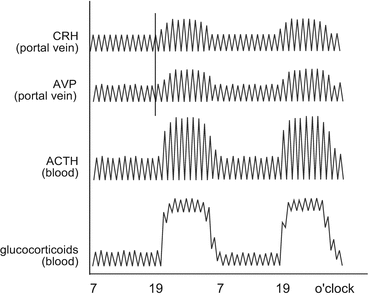
Fig. 11.2
Pulsatile and circadian activity of the hypothalamic–pituitary–adrenal axis. The pulse shift from corticotropin-releasing hormone (CRH) to arginine vasopressin (AVP) is indicated by the vertical bar. The amplitude changes in a circadian manner. Following the pulsatile CRH/AVP release, pituitary adrenocorticotropic hormone (ACTH) is released in pulses and stimulates adrenal synthesis and release of glucocorticoids . Elevated AVP/CRH pulses in the evening and during the night result in markedly enhanced glucocorticoid levels still oscillating with a frequency of 20 min (Redrawn from Chrousos 1998)
The central role of the mutual interaction of the paraventricular nucleus and locus coeruleus has been questioned in recent years. In the last 10 years, several authors have reported on specific reactions to stressors which cannot be reconciled with the definition of Selye.
One aspect of stress regulation is the action of glucocorticoids in the brain. The patterns is most complex. Corticosterone and cortisol were observed in brain; released from the adrenal gland, they are transported by corticosteroid-binding globulin or unbound (may be attached to albumin). Whether corticosteroid-binding globulin can cross the blood–brain barrier has not been analyzed; steroids, as far as we know, are able to do so. In the periventricular tissue around the blood capillaries, 11-β-hydroxysteroid dehydrogenase (11β-HSD) type 2 is strongly expressed, converting cortisol into the inactive 11-deoxycortisol (see Fig. 6.21).
In many animal species, corticosterone (Fig. 6.21) instead of cortisol is the active glucocorticoid. This is not converted by 11β-HSD type 2 . Corticosterone, too, binds to corticosteroid-binding globulin, but with a somewhat lower affinity. Furthermore, cortisol is removed from cells of the blood–brain barrier by the multidrug resistance P-glycoprotein ; this multidrug resistance protein serves, in general, to move toxic substances. Obviously, cortisol is regarded as something foreign, whereas corticosterone is not. Thus, there are two mechanisms which favor the use of corticosterone in the brain.
Corticosterone binds preferentially to mineralocorticoid receptor (MR ) and with reduced activity to glucocorticoid receptor (GR ); both nuclear receptors are transcription factors. To make the situation even more complex, hippocampus cells express another 11β-HSD, of type 1, which converts corticosteroid to cortisol, which, again, prefers to bind to GR (Kloet 2003). Whether 11β-HSD type 1 is widely distributed and whether its expression is required for glucocorticoid activity in the central nervous system (CNS) has not been reported.
These findings still leave open the question whether cortisol is active in the brain. Corticosterone might bind to MR and GR. Maintaining homeostasis is achieved via MR, whereas GR-induced activities seem to facilitate recovery after disturbances of homeostasis (Kloet 2003).
11.2.2 Calcium Metabolism
Since there are closed blood circuits, the free calcium concentration in blood is a fairly exact 1 mmol/l. This is exactly the calcium concentration in saltwater, from which it is inferred that all organisms are derived from saltwater organisms.
In humans calcium is taken up with the food (1 in Fig. 11.3), brought into the blood, and stored there complexed with albumin (2 in Fig. 11.3); albumin–calcium serves as a buffer for the blood calcium concentration. Calcium from blood is incorporated into bone (3 in Fig. 11.3), or filtered from blood by the kidney (4 in Fig. 11.3) and if required resorbed from the primary filtrate (5 in Fig. 11.3).
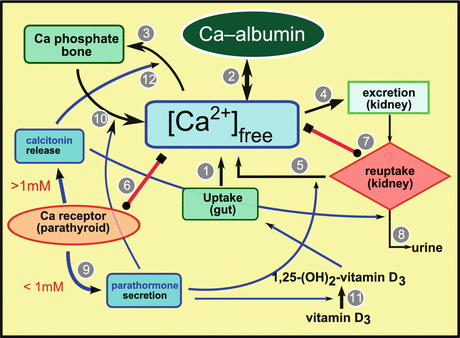
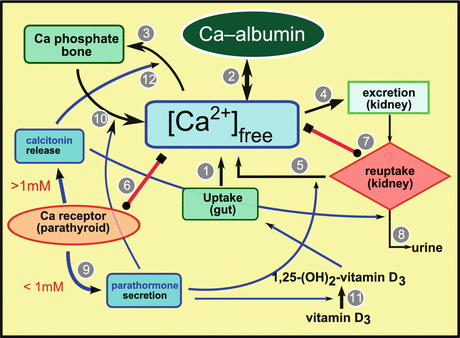
Fig. 11.3
Major components of calcium metabolism
The calcium level is controlled by calcium concentration sensing receptors in the surface of parathyroid cells (6 in Fig. 11.3) or kidney cells (7 in Fig. 11.3) . The sensor is a heptahelical G-protein-coupled membrane receptor . When the sensor is stimulated by elevated calcium concentrations, it activates phospholipase to enhance the level of inositol trisphosphate (IP3); by this messenger, resorption in the kidney is inhibited and filtered calcium is secreted in urine (8 in Fig. 11.3). In the parathyroid gland, IP3 inhibits the synthesis of parathormone (9 in Fig. 11.3). If the calcium secretion leads to a deprivation of calcium, IP3 release is blocked and resorption in the kidney and parathormone synthesis in the parathyroid gland are induced.
By parathormone, the level of available calcium is increased by desorption from bone (10 in Fig. 11.3). Simultaneously, the conversion of 25-hydroxyvitamin D3 to 1,25-dihydroxyvitamin D3 (calcitriol) (11 in Fig. 11.3) is triggered, which then allows uptake of calcium in the gut and its transport through the gut wall.
The antagonist of parathormone is calcitonin ; its formation is triggered by elevated (greater than 1 mM) calcium levels. This hormone blocks resorption in the kidney and facilitates calcium incorporation into bone (12 in Fig. 11.3).
11.3 Regulation of Reproduction
Progeny and reproduction are among the most important characteristics of life. Since the debut of science, the study of these phenomena has occupied investigators. The differences between women and men have been obvious since primeval times, and children learn the functions of sexual organs at the latest in their first sex education at school.
The influence of hormones on reproduction is common knowledge to any woman taking or having taken the pill. The role of hormonal regulation during the menstrual cycle is less well known. The hormonal control of male reproductive capacities is almost unknown. The male sex hormone testosterone receives more attention when its abnormal levels are used to help defend a person accused of rape in court.
11.3.1 Regulation of GnRH
Reproductive rhythms are controlled via GnRH. Sexual activity in animals is allowed when sufficient food is available and the social fabric allows it; in many animals, a seasonal rhythm maintains control of sexual activity. Additionally, only pulsatile GnRH release induces pulsatile release of gonadotropins, which in turn regulate gonadal activity. These observations are reflected in the regulation of GnRH; neurotransmitters such as NPY, endorphins, CRH, and galanin are messengers of these environmental conditions which by controlling GnRH formation and release allow reproduction.
The perikarya of the GnRH neuron s are present in the hypothalamus, mainly in the preoptic area and in the median eminence, and in addition in the lamina terminalis and septal area (Fig. 11.4). The former have been shown to be innervated strongly by other neurons, while the latter are only sparsely connected with other neurons.
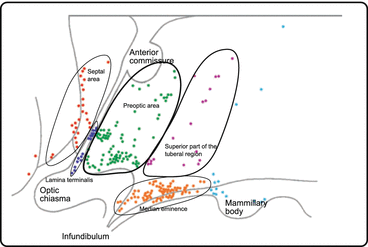
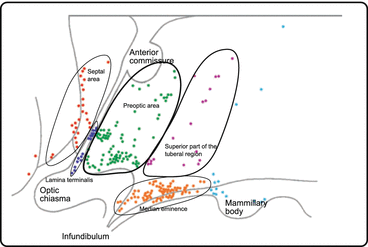
Fig. 11.4
Gonadotropin-releasing hormone (GnRH)-secreting neurons in the human hypothalamus. The perikarya of GnRH neurons are in the labeled areas. GnRH neurons are strongly innervated by other neurons, with the exception of those in the septal area (From Dudas and Merchenthaler 2006)
The following neurotransmitters influence GnRH neurons (Fig. 11.5): γ-aminobutyric acid (GABA), NPY, substance P, endogenous opiates such as endorphins and leu-enkephalin, CRH, galanin, catecholamines (dopamine and noradrenaline), and neurotensins (Dudas and Merchenthaler 2006) (Fig. 11.5).
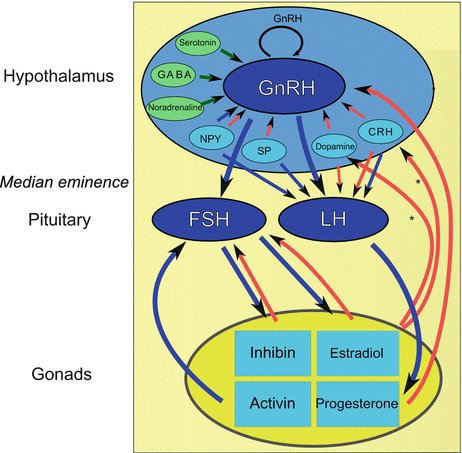
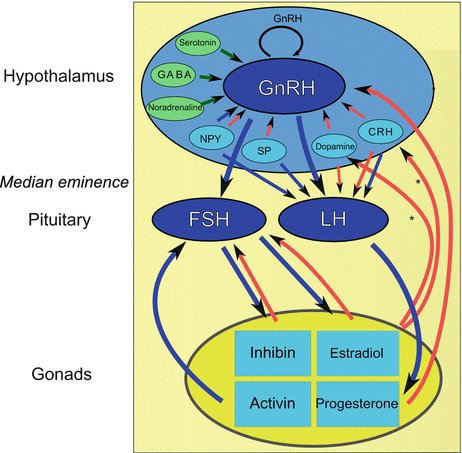
Fig. 11.5
Hormonal interplay for successful reproduction. Blue arrows amplifying endocrine secretion, red arrows mitigation of endocrine secretion, green arrows neurotransmitter activity [tuberoinfundibular dopaminergic neurons have been found to express estrogen receptors and may thus exert feedback control on gonadotropin-releasing hormone (GnRH) release], asterisks indicate that other neurons are potentially estrogen receptor positive and may participate in this feedback control, CRH corticotropin-releasing hormone, FSH follicle-stimulating hormone, GABA γ-aminobutyric acid, LH luteinizing hormone, NPY neuropeptide Y, SP substance P (From Dudas and Merchenthaler 2006)
Tuberoinfundibular dopaminergic (TIDA) neurons, which release dopamine, are important control elements for GnRH release. In those areas where TIDA neurons are found, there are also many NPY, galanin, endorphin, and substance P neurons, whereas CRH neurons are mainly located in the infundibulum and the preoptic area (Dudas and Merchenthaler 2006). TIDA neurons, furthermore, express estrogen receptor, allowing gonadal feedback inhibition of GnRH release (Mitchell et al. 2003). The latest addition to the list of GnRH regulators is kisspeptin (Messager et al. 2005).
Suppression of reproductive activity as a consequence of stress has been established in mammals. The central and peripheral stress systems are thought to play the prominent role: CRH directly suppresses GnRH release via synaptic contact of CRH axons to dendrites of GnRH neurons in the medial preoptic nucleus. There are differences between rodents and primates. Endogenous opiates (from proopiomelanocortin) can mediate several CRH effects, in part with respect to the menstrual cycle, to the species, or to the sex. Cytokines from the CNS are equally involved in GnRH regulation: IL-1 inhibits GnRH neuron activity and reduces GnRH synthesis and release. These IL-1 effects are mediated in part by endogenous opiates (endorphins and enkephalins) and by central prostaglandins.
11.3.2 Regulation of the Menstrual Cycle
Regulation of the female menstrual cycle occurs in four organs: in the hypothalamus, in the pituitary, in the ovaries, and in the uterus. We are talking about the hypothalamic–pituitary–gonadal axis .
The first essential variable indispensable to reproduction is pulsatile release of GnRH in the hypothalamus . There are only some thousand GnRH-secreting neurons in the mediobasal hypothalamus and in the preoptic nucleus. Triggered by γ-aminobutyric acid (GABA ) and owing to mutual axonal connections of GnRH neurons, a coordinated secretion in the median eminence occurs. Whether galanin coexpressed in many GnRH neurons plays a role in GnRH secretion is an open question. GnRH secretion is reduced or entirely blocked by stress and its mediator CRH.
Without pulsatile release—that is, with continuously elevated or missing GnRH levels—LH/FSH release is efficiently blocked. The molecular mechanisms for this effect are unknown. Failure of GnRH receptor recycling and thus lack of cell surface expression of the GnRH receptors might explain short-term unresponsiveness of gonadotropic cells to GnRH. A possible block of synthesis has not yet been proven, but may be the simplest explanation for long-lasting LH/FSH suppression.
Because of this LH/FSH deficiency, continuous dosing of GnRH or GnRH analogs has been used for temporary contraception.1
Following pulsatile release of GnRH into the portal system of the infundibulum, LH and FSH release is induced in the pituitary gonadotropic cells.
FSH initiates maturation of cohorts of primordial ovarian follicles into primary and secondary follicles (meiosis) . LH stimulates in theca cells around the follicles synthesis of testosterone, which after diffusion into the follicles is converted into estradiol . The largest follicle synthesizes amounts of estradiol which inhibit pituitary FSH release. In cows, and possibly in general, this follicle’s growth is no longer dependent on FSH, but the growth of the other follicles still is, and thus they degenerate on lack of FSH. In addition to estradiol, the follicular fluid contains a protein heterodimer: inhibin . In concert with estradiol, inhibin blocks specifically pituitary FSH release, but not LH release, although both LH and FSH have been found together in the secretory granules. Inhibition is obviously due to blocking of FSH synthesis. Follistatin is also involved in this process.
Although only the dominant follicle grows further, androstenedione , testosterone , 17-hydroxyprogesterone , and estradiol concentrations in blood increase steadily. Shortly before ovulation there is a LH surge . Finally, the follicle bursts and liberates together with the oocyte its hormonal content. The estradiol stimulates growth of the uterine endometrium. This allows nidation of the fertilized egg within a restricted time window and which may grow into a new individual.
The corpus luteum develops from the remnants of the burst follicle. The corpus luteum provides progesterone , which inhibits degeneration and rejection of the uterine endometrium. Progesterone together with estradiol from the former follicle decreases hypothalamic GnRH release. This progesterone synthesis is maintained for only a few days and finishes if fertilization does not occur (see the next paragraph) after 7–8 days. As a consequence, blood supply to the endometrium is blocked, and the latter degenerates until it is rejected during the following menstruation. Without inhibition by estradiol and progesterone, hypothalamic GnRH release is reinitiated, FSH is made in the pituitary, and the next wave of follicles start to grow. The cycle restarts.
In the case of fertilization, the fertilized egg starts to divide while still in the ovarian duct. After the 32-cell stage , cells fuse to the morula , which gives rise to the blastocyst , with an outer layer of trophoblastic cells and the inner cell mass . The fetus develops from the inner cell mass, and the placenta develops from the trophoblastic layer. The trophoblastic cells perform two roles: they mediate nidation into the uterine endometrium and they synthesize the pregnancy hormone choriogonadotropin . With this choriogonadotropin, progesterone synthesis in the corpus luteum is maintained, progesterone levels remain elevated, and degeneration of the endometrium is inhibited. As long as trophoblastic cells of the blastocyst and later in the placenta secrete choriogonadotropin, progesterone synthesis in the corpus luteum is ensured. In addition, progesterone blocks pulsatile GnRH release from the median eminence and LH/FSH release from the pituitary.
11.3.3 Puberty
Men and women are not fertile immediately postpartum. The reproductional competence is acquired later in life, in most individuals after the 11th to 13th birthday. Pituitary hormonal release and gonadal hormonal release in the newborn drop off within a few weeks . Menarche, the time of first ejaculation or first menstruation, is in the middle of a long period of sexual maturation starting with enhanced adrenal activity at the age of about 8 years, called adrenarche . First DHEA and DHEA sulfate secretion is enhanced, then 1–2 years later androstenedione secretion is enhanced, but the reasons for this change are still unknown. The formation of these androgens starts the growth of primary and secondary sexual organs and the development of pubic hair.
Up to puberty no pulsatile LH or FSH is measurable. The earliest measurable parameters are nightly FSH pulses strongly dependent on GnRH pulses . These GnRH pulses are induced centrally without any gonadal costimulation since puberty occurs in individuals without functional gonads. About 1 year after the first occurrence of FSH pulses, LH pulses can be measured. These FSH or LH pulses are not yet regularly timed and of constant amplitude as in adults, but are relatively rare and with largely varying frequency. As a reaction to these gonadotropin pulses and because of the development of Leydig cells in boys and the first growing ovarian follicles in girls, testosterone and estrogen levels in blood increase and they become measurable. Mood changes are explained by these irregular gonadotropin pulses and thus changing steroid levels. The first FSH pulses induce only incomplete follicular development, and ovulation does not yet occur. In the course of puberty, pulses are more regular, follicle development reaches later stages, and finally the first ovulation occurs. Menarche is reached.
In boys, the first ejaculation is not at the end of puberty, since regular GnRH pulses and coupled LH and FSH pulses are not as frequent and regular as in men, where about 18 pulses per day is normal. Enhanced FSH release forces the formation of seminiferous tubules. Under the influence of LH, precursor cells develop into Leydig cells, which mainly release testosterone.
The enhanced testosterone formation coinciding with enhanced growth hormone (GH) levels induces strong mood changes: the teenager shows—compared with childhood—an enhanced aggressive behavior although the environment, obviously, did not change: the “only” change is the maturation of the endocrine system.
Apart from the hormones of reproduction, other hormones, especially GH, insulin, and insulin-like growth factor (IGF) 1, are produced in greater amounts. With GH levels reduced, puberty is retarded.
11.3.4 Menopause
After the arrival of sexual competence, men and women are fertile for quite some time, until in women at the age of about 50 years menstruation fails to occurs. When pregnancy can be excluded—for example, in the absence of choriogonadotropin—then menopause has been reached. Since many follicles mature during the follicular stage of the menstrual cycle, with one or perhaps two arriving at ovulation, one assumes that the storage of egg cells/follicles capable of growing has been emptied. In 35 years there are about 13 cycles per year; in total 455 cycles. With 20 growing follicles per cycle, about 9,000 follicles have matured. Why the rest of the about 100,000 egg cells counted at birth do not mature is not known. About 220 follicles should mature per cycle in order to expend 100,000 primordial follicles within 455 cycles.
In men, sperms are not formed antepartum, but are first made during puberty. As long as the hormonal endowment in a man of 70 years—an age at which women are normally no longer fertile–allows there to be sufficient LH, FSH, and testosterone production, then the man is still fertile. For men andropause is presumed to be equivalent to menopause in women, but a fixed time point cannot be estimated.
Gamete development differs remarkably in men and women:
By two meiotic divisions, four sperms arise, but a single haploid egg arises. Those chromosomes not utilized in women, which are called polar bodies, are removed from the egg.
The first meiotic division happens in women already in utero; the second one happens after ovulation.
In men both meiotic divisions occur during sperm development in the adult testis.
The frequency of genetically arising malformation in newborns rises with the age of both parents. There is no evidence that there is a sex bias in gamete errors. Obviously, several primary factors are balanced:
Advantages in men: a single sperm of many fertilizes the egg, errors are diluted.
Disadvantages in men: errors might be acquired in sperm stem cells during the long fertilization period.
Advantages in women: after the first division an enrichment of reduplication mismatches does not occur; three quarters of the chromosomes will be removed.
Disadvantages in women: a single egg is not selected from many other egg cells; once an error is there, it will be propagated.
In the past only the mother’s age was reflected on when arguing for genetic counseling, but today the father’s age is also taken into account.
11.4 Glucose Metabolism
11.4.1 The Origin of Blood Glucose
The organism derives glucose from three different sources: from food, from glucose stores, and from glucose synthesis :
1.
Glucose from food. Glucose is taken up from the gut by a transporter protein which simultaneously takes up two sodium ions per glucose molecule (sodium–glucose cotransporter 1; Lee et al. 1994). The same transporter takes up other sugars as well.
2.
Glucose storage. When in excess, glucose is stored as glycogen mainly in the liver. When glucose is needed, these stores can be emptied and glycogen can be converted into free glucose.
3.
Gluconeogenesis. In an inverted process of glucose oxidation to CO2, glucose can be newly generated from intermediates using energy. Gluconeogenesis mostly occurs in the liver.
11.4.2 Regulators and Control Variables
Insulin is the characteristic hormone of glucose regulation (see Sect. 4.7). Its antagonist is glucagon. Both hormones are released from specialized cells of the pancreas (see Sect. 10.6).
The control variable of glucose metabolism (Fig. 11.6) is the glucose concentration in blood !. Dependent on the glucose concentration, there are two stages: fasting and sated. The threshold is 5 mM glucose. Below 5 mM there is fasting, above 5 mM, there is satiation.
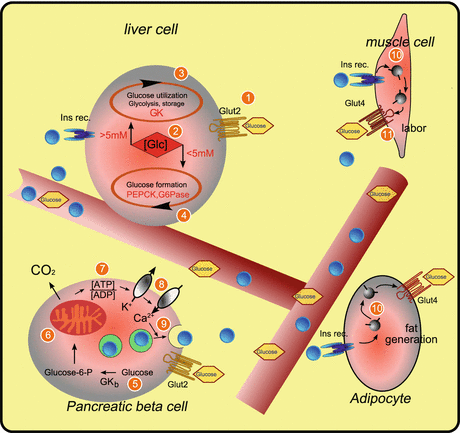
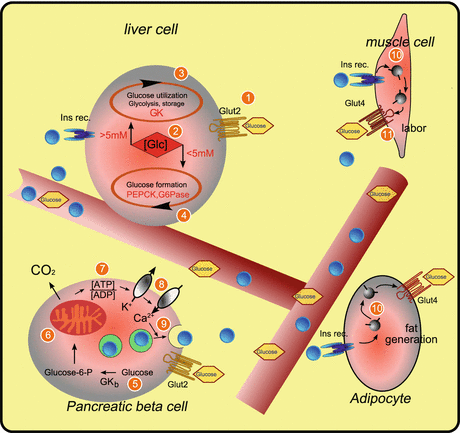
Fig. 11.6
Elements of glucose metabolism. Blue spheres insulin, GK glucokinase, GK b glucokinase content of β cell, Glc glucose, glucose-6-P glucose 6-phosphate, Glut glucose transporter, G6Pase glucose 6-phosphatase, Ins rec. insulin receptor, PEPCK phosphoenolpyruvate carboxykinase
11.4.3 Glucose-Dependent Gene Expression in the Liver
The liver switches between the two states depending on the glucose concentration: in the sated state, glucose is removed—by catabolization or by storage as glycogen; in the “fasting” state, glucose is synthesized or is provided by emptying glycogen stores . The regulating enzyme is the intracellular glucokinase , which converts glucose to glucose 6-phosphate (Glc6P), which is then used further. Intracellular regulation is possible owing to the high availability of glucose transporter 2 on the liver cell membrane (1 in Fig. 11.6).
On other cells there are glucose sensors from the family of glucose transporters: sodium–glucose cotransporter 3 (Diez-Sampedro et al. 2003). Owing to the activity of the sensors, there is a gene expression switch from glucose utilization to glucose production and vice versa (2 in Fig. 11.6). In the liver, at high glucose concentration, glucokinase expression is enhanced and thus Glc6P production is triggered, which leads either to catabolism or to glucose storage (3 in Fig. 11.6). When the glucose concentration is low, Glc6P is made available from stores or by gluconeogenesis , and the glucose produced following its cleavage is secreted into the circulation (4 in Fig. 11.6).
The rate-determining enzyme of glucose anabolism is phosphoenolpyruvate carboxykinase , whose expression is stimulated at low glucose concentration. For the conversion of Glc6P into glucose, glucose 6-phosphatase is required, and this also has a glucose-concentration-dependent expression.
Insulin has a role in glucose storage in the form of glycogen.
11.4.4 Glucose-Dependent Insulin Secretion in the Pancreas
Insulin is released in the pancreas at high glucose concentration:
1.
Glucose is gated into pancreatic β cells by glucose transporter 2 .
2.
3.
Since ATP is gained in the catabolism, the cytosolic ATP-to-ADP ratio is increased (7 in Fig. 11.6).
4.
At an elevated ATP-to-ADP ratio, potassium ions are transported from the cell via a potassium channel (8 in Fig. 11.6).
5.
This generates a voltage change at the membrane and induces the opening of calcium channels and an influx of calcium ions.
6.
These in turn induce fusion of secretory insulin vesicles with the cell membrane (9 in Fig. 11.6), and the vesicle content is released. The released insulin acts as a hormone in the regulation of blood glucose.
11.4.5 Insulin-Dependent Procedures
In the liver, insulin stimulates Glc6P storage as glycogen . Additionally, insulin induces release of amino acids from liver cells to be used in muscle cells (insulin triggered) for protein synthesis .
In muscle cells, insulin triggers, mediated by insulin receptor (10 in Fig. 11.6), gating of preformed glucose transporter 4 from intracellular vesicles to the membrane, thus stimulating glucose uptake (11 in Fig. 11.6). The glucose taken up is used to support muscle activity.
Similarly, insulin triggers uptake of glucose into adipose cells mediated by glucose transporter 4, where glucose is used for fatty acid synthesis.
11.4.6 Glucagon and Blood Glucose Level Increase
Whereas at elevated glucose concentration insulin is secreted from β cells, at low glucose concentration pancreatic α cells secrete glucagon (see Sects. 4.7 and 10.6). In liver cells, glucagon stimulates protein kinase A , the key enzyme in gluconeogenesis and glycogen catabolism, both reactions enhancing the level of available glucose.
11.5 Appetite and Hunger
Human ingestion is controlled consciously and unconsciously (Fig. 11.7). We are able to control our eating behavior only in part: it is common experience that bursts of ravenous appetite send our good intentions packing. An autonomous regulation separated from conscious control (again in part) is active. The purpose of this control is a long-lasting constancy of body weight and therefore safeguarding of the individual’s survival. To achieve this, food and energy uptake and energy consumption have to be balanced.
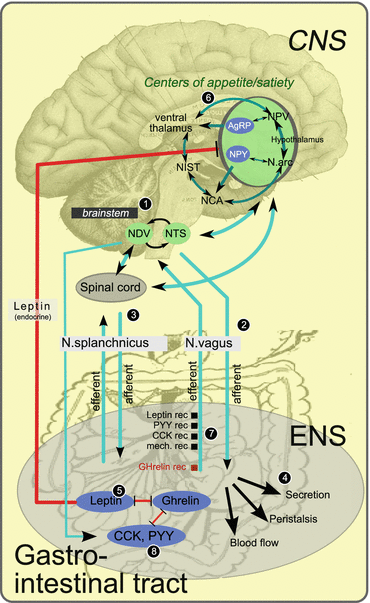
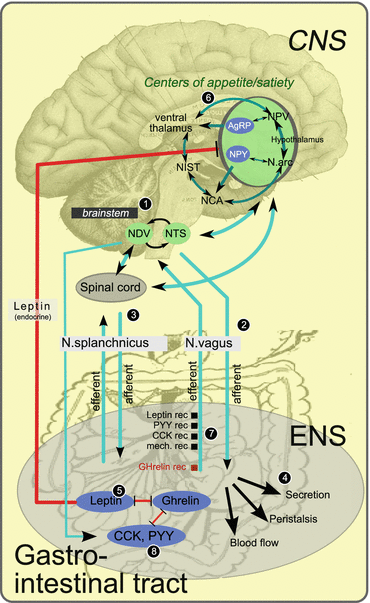
Fig. 11.7
Brain–belly interplay: regulation of appetite and hunger. Red lines inhibitory endocrine actions, blue-green lines control by nerves and synapses, AgRP agouti-related peptide, CCK cholecystokinin, CNS central nervous system, ENS enteric nervous system, mech. rec mechanoreceptor, N.arc arcuate nucleus, NDV dorsal motor nucleus of the vagus nerve NCA central nucleus of the amygdala, NIST bed nucleus of the stria terminalis, NPV paraventricular nucleus, NPY neuropeptide Y, NTS nucleus of the solitary tract, PYY peptide tyrosine tyrosine, rec receptor (From Konturek et al. 2004; Gray 1918, Fig. 715)
In this control system comprising the brain, adipose tissues, and gastrointestinal (GI) tract, hormones from the GI tract, from adipocytes, and from neurons are involved. The presentation of these circuits is limited to an overview owing to space restrictions; in addition, many details have not been fully and sufficiently analyzed.
Konturek et al. (2004) reviewed the regulation of appetite and body weight .
11.5.1 Central Nervous System
In the CNS (Fig. 11.7) the nucleus of the solitary tract regulates gut secretion, gut movement, and blood supply. Satiety and appetite are controlled in different centers, mainly in the arcuate nucleus and the paraventricular nucleus, as well as in the ventromedial and lateral hypothalamus. Also involved are the central nucleus of the amygdala , the interstitial nucleus, and the ventral thalamus .
11.5.2 Parasympathetic Fibers of the Vagus Nerve
Starting from the brainstem (1 in Fig. 11.7), the vagus nerve interconnects multiple organs of the GI tract. About 20,000 nerves connect to different cells of the GI tract.
11.5.3 Sympathetic Fibers of the Splanchnic Nerve
Synapses of the splanchnic nerve connect the GI tract with the brain (3 in Fig. 11.7).
11.5.4 Enteric Nervous System
A specialized nervous system is generated by about 100 million nerve cells of the enteric nervous system . These nerves stimulate and inhibit the entire GI events in an intrinsic way, whereas the vagus nerve and the splanchnic nerve interconnect the GI system with the brain. Secretion of digestion enzymes , salts, or hydrochloric acid, peristalsis , and the circulation are controlled by these nerves (4 in Fig. 11.7).
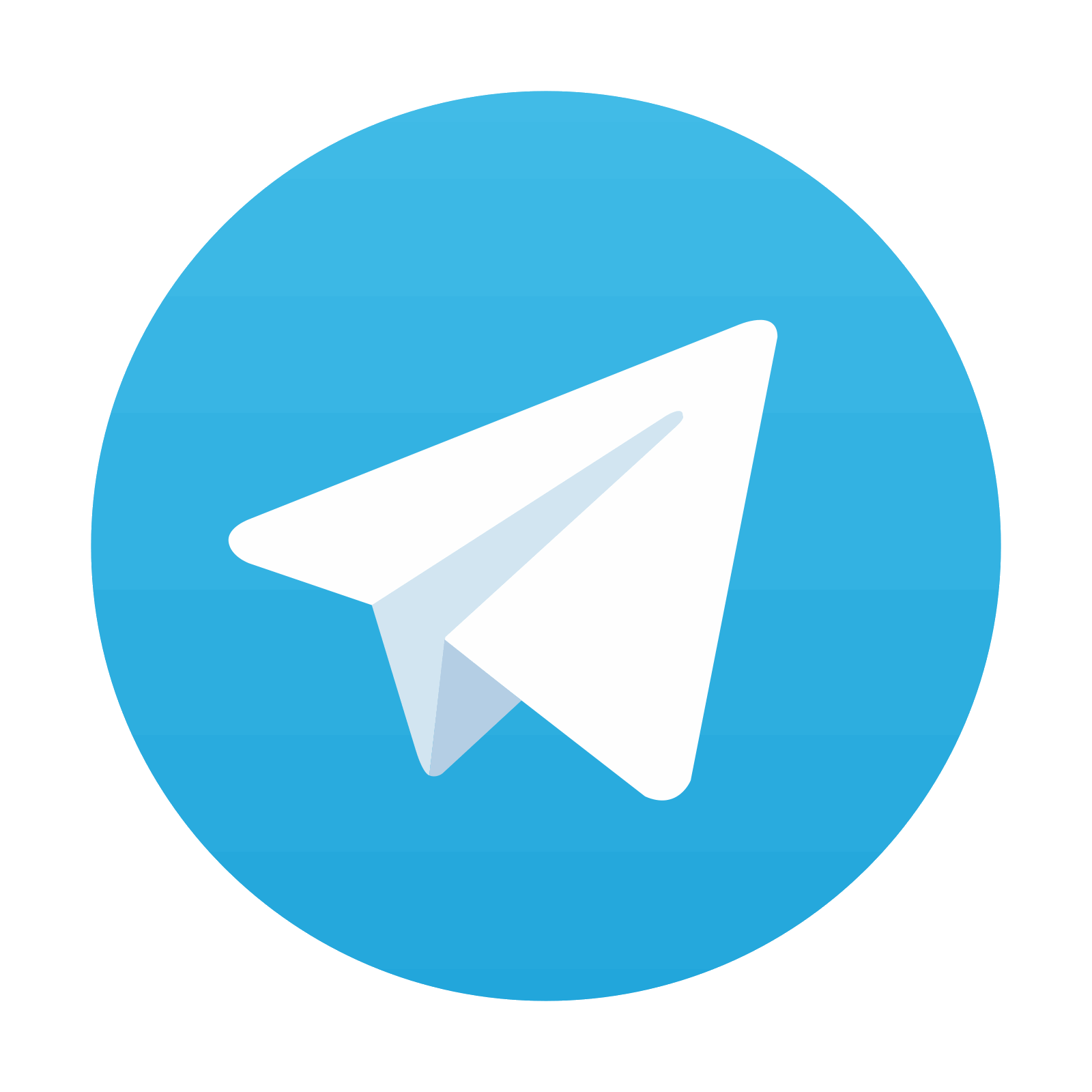
Stay updated, free articles. Join our Telegram channel
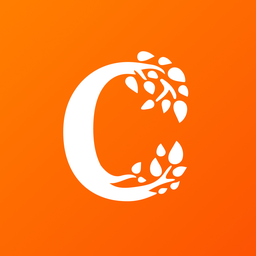
Full access? Get Clinical Tree
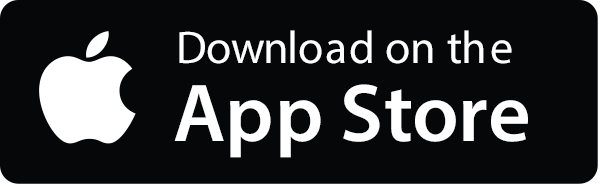
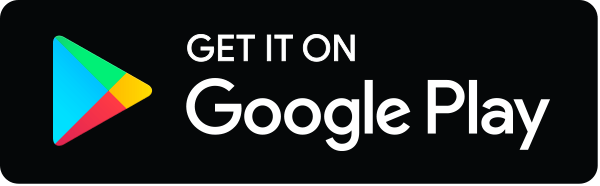