Sample patient immobilization and field set-up for whole-brain radiotherapy (WBRT) via opposed lateral fields. (a) Custom headholder with field shape outlined on mask; (b) multileaf collimator outline (dark blue lines); and (c) radiation dose distribution – starting from upper right-hand corner and moving clockwise: 3D rendering of dose distribution and isodose lines for sagittal, coronal, and axial cross-sections.
Highly conformal partial brain irradiation
In treating primary brain tumors, we typically utilize 3D conformal radiotherapy (3D-CRT) or intensity-modulated radiotherapy (IMRT). In 3D-CRT, radiation is delivered via several beams directed toward the center of the target (the isocenter) with the aperture of each beam shaped to fit profile of that target. When treating brain lesions with 3D-CRT, our practice is to use five or six non-coplanar beams, often of different energies, as this achieves very good conformality for regularly shaped lesions with excellent dose homogeneity. In IMRT, the beam aperture is continually adjusted (modulated) such that a differential radiation dose may be delivered to different portions of the target. This “dose painting” permits irregularly shaped targets in close proximity to critical normal tissues to be adequately covered by the desired dose of radiation while sparing the adjacent normal tissue. Depending on the size and location of the lesion, five to seven static beams or two to three volumetric-modulated arc IMRT plans may be used.
IMRT requires sophisticated computer-based optimization to achieve the desired dose distribution and specialized quality assurance (QA) to ensure that the plan is correct and executable. 3D-CRT plans can be generated quickly and can readily be delivered, utilizing straightforward QA techniques. However, it is difficult to conform the dose to follow concave surfaces, e.g., a lesion in the anterior temporal lobe abutting the brainstem and anterior visual pathways (AVPs). In these situations, IMRT offers the advantages of superior dose distributions at the expense of higher planning effort, more complex QA, and often lengthier treatment delivery times. Representative radiation dose distributions for IMRT for a brain lesion are shown in Figure 3.2.
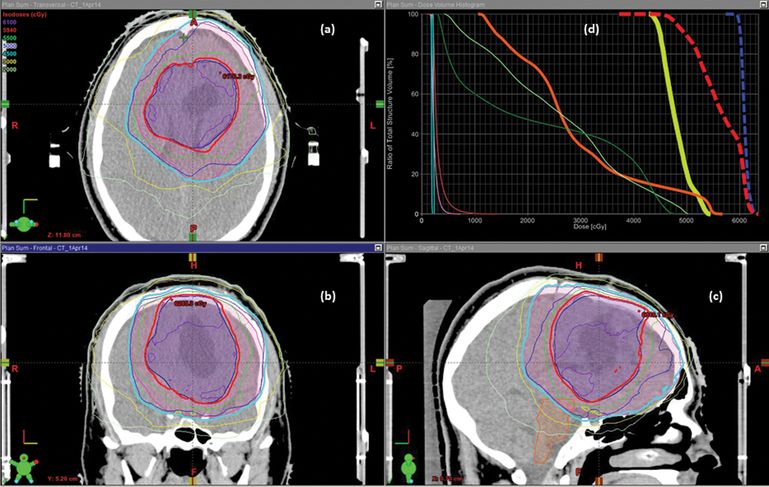
Sample dose distribution and dose–volume histogram for intensity-modulated radiotherapy (IMRT) via dynamic conformal arcs to a left frontal glioblastoma multiforme postresection. Starting from upper left-hand corner and moving counterclockwise: 2D rendering of dose distribution and selected structure contours for (a) axial; (b) coronal; and (c) sagittal cross-sections, with particular note to clinical target volume (CTV) primary (pink volume), CTV boost (blue volume). 5940 cGy isodose line (thick red line) and 4500cGy isodose line (thick cyan line). (d) Dose–volume histogram showing cumulative dose for both primary and boost plans to CTV primary (thick blue line), CTV boost (thick red line), optic chiasm (thick yellow line), brainstem (thick orange line), optic nerves (thin light and dark green lines), eyes (thin pink and red lines), and lenses (thin light blue lines).
Effectively executing these plans requires that the patient’s head be held in a reproducible, stable position and that the head/tumor position be verified and, if necessary, adjusted as described below. Appropriate application of image-guided radiotherapy (IGRT) ensures that these highly conformal partial brain irradiation plans can be successfully executed, i.e., the target lesion can be covered and the dose to normal brain structures minimized without fail. Consequently, IGRT will minimize margin expansions about the target that have historically been employed to account for set-up error and position deviation.
Stereotactic radiosurgery (SRS)
In SRS of brain lesions, a high dose of radiation is delivered in a single fraction or a few fractions with rapid dose fall-off from the periphery of the target lesion into the surrounding normal brain tissue. While a variety of disparate radiotherapy systems, e.g., GammaKnife (Elekta), CyberKnife (AccuRay), Novalis Tx (Varian Medical Systems and BrainLab), and TrueBeam STX (Varian), are utilized in intracranial SRS, all share some features, as described in the attached consensus definition for radiosurgery (Table 3.1).9 All systems require and exhibit exquisite accuracy (<1 mm deviation) for patient immobilization, target localization, and dose delivery. Typically, a patient is immobilized with either a semi-rigid, custom-molded, removable head mask (Figure 3.3a) or a stereotactic head ring fixed to the patient’s skull. Target delineation is carried out using fine-cut CT scans (1–1.25 mm slice thickness) fused with contrast-enhanced magnetic resonance imaging (MRI) images and occasionally functional imaging modalities, such as positron emission tomography.
|
Adapted from G. Barnett et al. J Neurosurg 2007.9
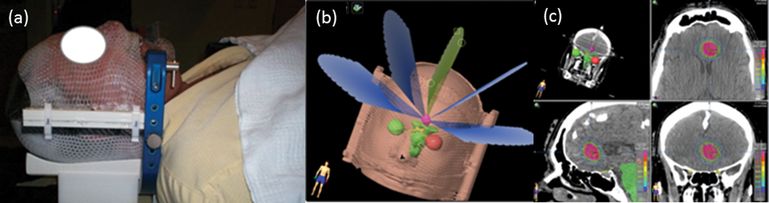
Sample patient immobilization and field set-up for stereotactic radiosurgery (SRS) via dynamic conformal arcs to a medial left frontal brain metastasis. (a) Custom U-frame mask; (b) multiple arc paths; and (c) radiation dose distribution – starting from upper left-hand corner and moving clockwise: 3D rendering of dose distribution and isodose lines for axial, coronal, and sagittal cross-sections.
In a collimator-based linear accelerator system, such as the Novalis Tx and TrueBeam STX employed at our institution, a typical treatment plan for an ellipsoid lesion, such as a nodular recurrence of a malignant glioma, consists of three to five non-coplanar conformal arcs (as illustrated in Figure 3.3b), yielding the conformal dose distribution shown in Figure 3.2c. Multiple intensity-modulated beams are often used for treating irregularly shaped targets and/or those that are intimately associated with critical organs. The correlation between the patient geometry (and/or immobilization device geometry) and the treatment machine geometry is achieved through two different approaches: (1) matching the geometry of the immobilization device with the machine isocenter through dedicated measurement devices with the assistance of room lasers; and (2) matching planning/simulation images with treatment (or onboard) images (either 2D orthogonal images or 3D cone beam CT images) acquired using an imaging device mounted in the treatment room (or machine) while the patient is at the treatment position. Both approaches are able to achieve localization accuracy of about 1 mm. Radiation delivery consists of multiple beams intersecting at a single point (isocenter), shaped by fixed geometry cones and delivered in multiple arcs, dynamically conformal arcs continuously shaped by a multileaf collimator or multiple intensity-modulated static beams or dynamic arcs.
In the CyberKnife system, multiple collimated small-diameter beams are delivered to an intracranial lesion using a linear accelerator attached to a highly mobile robotic arm. In contrast, the GammaKnife system uses hundreds of gamma-ray sources (Co-60) precisely collimated to intersect at a single isocenter. This yields a small spherical or ellipsoid high-dose cloud whose diameter is determined by the size of the collimator opening. Dose fall-off from the cloud into the surrounding tissue is extremely rapid. Treatment of irregular and/or large targets is achieved by “packing” together multiple dose clouds, positioned in the target by precisely repositioning the stereotactic headframe with respect to the machine isocenter.
Basic principles of radiation therapy practice
The radiotherapy process
The guiding principle underlying brain radiotherapy is accurate delivery of radiation to a target to kill or, at least control, the underlying malignancy, while minimizing the radiation dose to adjacent normal tissues. Effectively and safely accomplishing these conflicting goals requires quantitative visualization and localization of the target lesion, complex radiation plans, management of the target position throughout treatment, and robust QA. A schematic of the process for planning and delivering intracranial RT is shown in Figure 3.4.
Initial consultation
Selection of the appropriate treatment requires a thorough history, physical exam, and review of relevant imaging studies, pathology, and laboratory results, preferably in a multidisciplinary setting. Developing an integrated treatment plan requires discussions with the neurosurgeons and neuro-oncologists prior to this visit, as well as close communication with the patient and family/caregivers during and after the consultation. The diagnosis of a brain tumor is often an overwhelming experience, often complicated by physical, emotional, and financial upheaval, and it is essential that the radiation oncologist spend whatever time necessary to listen to the patient’s concerns. Most patients and their families/caregivers will not remember the details of the initial visits, no matter how calmly and clearly the facts are presented, and they must feel welcome to ask questions of the radiation oncologist, nurses, social workers, and other staff involved in their care at any time.
During the initial discussion on the treatment plan, it is important to describe what will be done, why it is being done, and how long the overall process and daily treatments will take. In addition, we find it useful to briefly explain how we will ensure that the treatment plan is correctly executed. In obtaining informed consent, we emphasize the risks and benefits, specific acute and long-term side effects, logistics, and uncertainties of treatment. In regard to this last point, we see few patients who expect us to guarantee outcome and eliminate uncertainty. However, it is a reasonable expectation that we will provide the competent and attentive care that will minimize risk and improve the outcome of their course of treatment.
Simulation
In this phase of the process, the patient is immobilized and imaged in the treatment position. The fundamental requirements for immobilization are that the device comfortably, reliably, and securely holds the patient in the treatment position over an entire session of stereotactic body radiation therapy (up to 1 hour.) Typically, the immobilization device consists of a custom-molded head-and-neck mask. This device also provides a precise, accurate correlation between the treatment machine and patient/target geometry, facilitating treatment planning and patient set-up during treatment.
After fashioning the mask, a high-resolution CT scan is typically performed to image the patient and treatment target, providing the precise, quantitative location of these structures in space. In addition, the simulation CT images are usually combined with MR images, which can provide superior visualization of soft-tissue lesions and anatomical structures. Our practice is to acquire a geometrically accurate, high-resolution contrast-enhanced T1-weighted MR series (typically a three-dimensional spoiled gradient recall echo [3D SPGR] series) during the simulation process. As simulation typically occurs 2–3 weeks following surgery, the high-resolution MR images will aid in delineation of critical anatomy, help differentiate immediate postsurgical artifacts from the target volumes, and identify any developing areas of disease.
Treatment planning
The first step in treatment planning is careful delineation of the target volume and normal tissue structures on the fused image set. Typically, this consists of the practitioner drawing the outline of the image on each slice of the image set (termed “contouring”), and these images are combined by the planning software to yield a 3D representation of the targets and normal anatomic structures. These images can be subsequently manipulated to add a margin to be treated or avoided around a structure, depending on the accuracy of imaging, precision of the treatment machine, judgment of microscopic extension of malignant cells beyond the visualized tumor, and the anticipated set-up error prior to treatment.
After contouring the structures and specifying the dose constraints, a treatment plan will be developed to effectively irradiate the target while meeting these constraints. Typically, a variety of treatment plans and techniques will be assessed, depending on the size and shape of the target, the prescription dose, the proximity and tolerance of the surrounding normal structures, and the specific treatment system selected. The choice of treatment plan will revolve around the number of radiation beams, beam energy, and the use of 3D-CRT versus IMRT. Treatment planning for partial brain irradiation is an iterative, labor-intensive process in which a plan is generated, the dose distribution critically reviewed by the involved physicians, and the plan discarded, optimized, or accepted.
Treatment delivery
As described above, a variety of devices may be used to deliver radiation therapy – each of which has specific advantages and limitations.
In all systems, the patient is securely positioned in the immobilization device on the treatment table and the position roughly adjusted using markings on the device or patient. In linear accelerators with multileaf collimators, orthogonal X-ray images and a cone beam CT are taken prior to treatment. Comparing the images acquired at planning and on the treatment table, the patient position is adjusted in the translational planes and rotational axes until the image sets exactly overlay one another in the region of interest.
Supportive care during radiotherapy
Patients with gliomas receiving a multi-week course of partial brain radiotherapy, usually with concurrent chemotherapy, require continual assessment and thoughtful support tailored to their medical and psychosocial situation. Patients experience fatigue, partial alopecia, and radiation dermatitis during radiotherapy, as well as disruption of their normal routine due to the requirement for daily radiotherapy, often with chemotherapy. We find that the weekly on-treatment visit with the radiation oncology physician and nurse is key to managing side effects, anticipating problems, and addressing the many concerns and questions that arise during treatment. Typically, during each weekly treatment check, we:
1. measure vital signs, with particular attention to changes in weight from week to week
2. review medications, ensuring that the patient is taking the correct dose of chemotherapy at the proper time each day
4. perform a focused physical examination
5. review weekly lab results (complete blood count, metabolic profile): a substantial drop in platelet count typically precedes frank temozolomide-induced thrombocytopenia and, when we observe a large decrease in platelet count (say from 250,000 to 125,000/mm3), we obtain a complete blood count at much shorter intervals
6. discuss the assessment of treatment to date, review the forward plan, adjust medications (typically steroids), and refer to other services (nutritional counseling, physical therapy, etc.), as necessary.
Patients and their families need to know that it is essential that they contact a member of their treatment team immediately if they have any questions or problems. We emphasize that we are available 24/7 and that they should not wait until the weekly treatment check to bring any issue to our attention.
Quality assurance
Highly conformal intracranial radiotherapy/radiosurgery is a complex and technically demanding process, requiring sophisticated equipment, attention to detail, and well-trained, experienced staff. A robust QA program, integrated into every aspect of the process with the commitment of all personnel, is absolutely required.10 A number of standard QA protocols have been developed and established for stereotactic body radiation therapy systems.11–13 However, an institution’s QA system must be tailored to the specific treatment system workflow and organizational culture, with continual measurement of errors and the application of corrective action to prevent any deviation.
Evidence-based practice
Primary malignant glioma
Radiation therapy following surgical resection of malignant gliomas has been a recommended component of the management strategy since the 1970s, as it improves overall survival compared to surgery alone. Walker et al.14 randomized patients with malignant glioma to one of four arms after surgery: chemotherapy (bis-chloroethylnitrosourea: BCNU) alone, radiotherapy (RT) alone, RT + BCNU, and best supportive care. Median overall survival was 14 weeks for best supportive care, 18.5 weeks for BCNU, 35 weeks for RT, and 34.5 weeks for RT + BCNU. All modalities using radiotherapy and/or chemotherapy provided statistically superior survival compared to postoperative supportive care alone. A subsequent study15 randomized 467 patients with malignant glioma to chemotherapy (lomustine: CCNU) alone, RT alone, RT + CCNU, or RT + BCNU. Median overall survival was 6 months in the CCNU-alone arm, 9 months with X-ray treatment (XRT) alone, 12.8 months with BCNU + XRT, and 10.5 months with CCNU + XRT. A meta-analysis16 of those and four other randomized trials revealed a significant survival advantage with the addition of radiotherapy to surgical resection compared to surgery alone (relative risk 0.81, 95% confidence interval 0.74–0.88, p < 0.00001).
With an objective of identifying an optimal dose for treatment of malignant glioma, Walker et al.17 analyzed the results from a variety of prospective trials, with doses ranging from 45 to 60 Gy. Median overall survival was 3.1 months without RT, 4.2 months with less than 45 Gy, 7 months for 50 Gy, 9 months for 55 Gy, and 10.5 months for 60 Gy. Survival was significantly different between groups receiving 50 versus 60 Gy (p = 0.004). A study18 of 474 patients with malignant glioma randomized to 60 Gy whole brain in 30 fractions vs. 45 Gy in 20 fractions found that median survival was significantly higher in the 60 Gy group (12 vs. 9 months, p = 0.007).
Trials to improve outcome by dose escalation using conventionally fractionated RT,19 hyperfractionation,20 brachytherapy,21,22 or a stereotactic radiosurgery (SRS)23 boost have not revealed a benefit to increasing dose beyond 60 Gy. For example, in RTOG 9305, 203 patients with glioblastoma were randomized to receive SRS versus no SRS prior to a course of conventional radiotherapy (a total of 60 Gy in 2 Gy daily fractions) and concurrent BCNU. No significant differences in survival (14.1 vs. 13.7 months with or without SRS), neurocognition, quality of life, or patterns of failure were found, with 90% of failures occurring at the treatment field in both arms. Thus, standard radiotherapy typically consists of 30 or 33 2.0 or 1.8 Gy daily fractions to a total dose of 59.4–60 Gy delivered over a 6–7-week period. However, there is evidence in elderly patients that treatment at a slightly higher dose per day for a significantly shorter period (e.g., 40 Gy delivered over 3 weeks) yields reasonable outcome,24 as discussed below.
While the entire brain was initially irradiated due to the concern about the widespread and insidious distribution of tumor cells throughout the brain, various trials showed no significant differences in outcome when the volume of brain irradiated was reduced.25,26 Pattern-of-failure analyses show that most failures occur with 2–3 cm of the enhancing lesion visualized on CT or MRI scan and that any distant failure is associated with a local failure.25,27 Thus, in order to avoid toxicity associated with whole-brain irradiation to 60 Gy, the current standard practice is to irradiate only the portion of the brain involved with tumor.
As described in detail in Chapter 4, in 2005 Stupp et al.28 reported the results of a European Organization for Research and Treatment of Cancer (EORTC) trial which randomized 573 patients with glioblastoma receiving radiotherapy (60 Gy in 2 Gy daily fractions) with or without the oral alkylating agent, temozolomide (TMZ). TMZ was administered once daily during radiotherapy and then for 5 days each month for the next 6 months. The addition of TMZ was associated with significantly improved overall survival (p < 0.0001), with median, 2-year, and 5-year overall survival 15 vs. 12 months, 27 vs. 11%, and 10 versus 2%, respectively.) Patients expressing lower levels of the enzyme responsible for repair of DNA damage, O6-methylguanine-DNA-methyltransferase (MGMT), exhibited a much more favorable response, though an improvement in survival was noted in the RT/TMZ arm even in those patients with unfavorable MGMT status.
Low-grade gliomas
In managing a patient with a newly diagnosed low-grade glioma, it is necessary to address several questions. First, should the patient be treated with radiation therapy, chemotherapy, or a combination of the two immediately, or should treatment be deferred until the time of progression? Second, if we believe that immediate radiation therapy is the treatment of choice for a patient with low-grade glioma, which radiation dose regimen best balances efficacy and toxicity? Finally, recognizing that a single approach does not apply to all low-grade glioma patients, it is equally important to ask, “Which treatment option is best for an individual patient?”
EORTC 2284529 addressed the question of immediate versus deferred treatment with radiotherapy. In this study, adults with low-grade glioma were randomized to receive 50.4 Gy radiotherapy starting immediately after they had recovered from surgery or biopsy versus initial observation with salvage treatment at the time of progression. While progression-free survival was superior in the arm receiving early radiotherapy (median 5.3 years vs. 3.4 years, p < 0.0001), overall survival was no different (7.4 years vs. 7.2 years, p = 0.87). In addition, there was no difference in the incidence of malignant transformation between the two arms. However, the study did not determine if there was a difference in the quality of life or neurocognition. This is a critical limitation, particularly in regard to selection of a treatment strategy, given the prolonged survival of most patients and the equivalent survival between the two arms.
The choice of a radiation dose regimen is somewhat clearer. EORTC 2284430 randomized 379 adults with low-grade glioma to 45 Gy versus 59.4 Gy, both at 1.8 Gy/fraction, and found no significant difference in either progression-free or overall survival. Similarly, in the Intergroup Trial of 203 adult low-grade glioma patients randomized to 50.4–64.8 Gy,31 overall survival did not favor either arm, with 5-year overall survival of 72% in the lower-dose versus 64% in the higher-dose arm. Radiation necrosis was somewhat higher in the arm receiving 64.8 Gy (5% vs. 2.5%), though results of the mini-mental status examination – an admittedly crude measure of neurocognitive function – appeared independent of total dose.32 As a result, we utilize a dose of 50.4–54 Gy in treating a low-grade glioma, as there is no apparent survival advantage and a risk of greater toxicity at higher doses.
In Radiation Therapy Oncology Group (RTOG) clinical trial 9802,33 251 adults with supratentorial low-grade glioma (all World Health Organization (WHO) grade II) were randomized to receive RT with or without concurrent procarbazine, lomustine, and vincristine (PCV) chemotherapy. The primary endpoint was overall survival, with progression-free survival a secondary endpoint. The rationale for RTOG 9802 was based on the observation that the addition of PCV to RT improved survival in anaplastic gliomas (RTOG 9402).34 Note that the study enrolled patients with unfavorable risk factors (age > 40 years old and/or less than gross total resection), with the implicit assumption that higher-risk patients might benefit from more aggressive therapy.
Comparing RT alone versus RT + PCV, median overall survival was 7.5 years versus not reached, respectively, and 5-year overall survival was 63% versus 72%, respectively. Neither difference was statistically significant. In contrast, progression-free survival improved with the addition of PCV (5-year progression-free survival 46% vs. 63%, p = 0.005). Both the progression-free and overall survival curves for the two arms diverged after approximately 2 years, and a post hoc analysis suggested that, for patients alive 2 years following initial treatment, the probability of surviving another 5 years was improved in the group receiving PCV (74% vs. 59%, p = 0.02). Updates to this study, presented in abstract form,35 show that the addition of PCV increased median progression-free survival and overall survival from 4.0 and 7.8 years to 10.8 and 13.3 years, respectively (p = 0.002 and 0.03.)
The question of differences in quality of life and neurocognition between these arms was not directly addressed in this study. Nonetheless, the much higher rates of grade 3/4 hematologic toxicity with RT + PVC versus RT alone (11% vs. 66%, p < 0.001) suggest at least some short-term disadvantage to PCV. However, the toxicity of PCV may be a moot point as temozolomide has largely supplanted PCV in the treatment of gliomas, due to its easier route of administration and perceived decreased toxicity. Phase II studies suggest that temozolomide is efficacious in the treatment of low-grade glioma and several cooperative group studies are testing the efficacy of temozolomide alone or with RT versus RT alone.
While RTOG 9802 enrolled “high-risk” patients, it is unclear if this definition precisely or accurately defines the best candidates for combined-modality treatment.36 As the authors point out, stratification of these patients by molecular characteristics may provide better insight into the benefit of this approach and, potentially, help predict the response to therapy. In summary, while this is a well-designed and executed study, maturation of the results is required before efficacy of combined PCV and RT versus RT alone in low-grade gliomas can be determined. Moreover, stratification based on molecular characteristics will be necessary, and it is not clear that we can directly extrapolate the results from 9802 to temozolomide-based regimens.
Recurrent gliomas
Given the extremely high rates of recurrence in malignant gliomas, improved treatment of recurrent disease is also a matter of great interest. Bevacizumab (BVZ) has been approved by the Food and Drug Administration for treatment of recurrent disease based on the results of phase II trials37–39 and SRS, alone40 or, particularly, in combination with bevacizumab,41–43 may offer benefits in this setting. However, randomized trials demonstrating the efficacy of SRS/BVZ have not been completed.
Essentially all recurrent primary malignant gliomas will have been treated with partial brain irradiation to a dose of around 60 Gy total in 1.8–2.0 Gy fractions, as discussed above. Transformed or secondary malignant gliomas should be considered for radiation using conventional regimens if not previously irradiated. In the setting of previous partial brain irradiation and recurrence within the volume of brain receiving an initial high dose of radiation (the most common scenario), it difficult to administer another “conventional” course of irradiation to the recurrent lesion and margin without risking adverse toxicity. Thus, hypofractionated stereotactic radiotherapy or radiosurgery to a limited volume is often employed. Alternatively, Marples et al.44 have proposed a novel approach to improve the therapeutic ratio and permit effective retreatment of large volumes, based on the principle of low-dose hypersensitivity and the application of pulsed irradiation.
Conceptually, SRS and hypofractionated stereotactic radiotherapy (HFSRT) offer beneficial features. First, the area requiring retreatment will be close to or within the area that has been manipulated during the initial surgery and treated to a high dose of irradiation. RTOG 9005 demonstrated that SRS of recurrent primary brain tumors could be performed with minimal morbidity and established the maximum tolerated dose for single-fraction SRS in this setting.45 Second, a short course of radiation has obvious logistic advantages over the much longer courses of radiation typically employed in primary treatment. Third, application of a high dose of radiation over a short period of time may evoke different mechanisms of tumor response and enhance tumor control,46,47 though this last point is controversial.48
However, the concept of SRS/HFSRT in the treatment of recurrent malignant gliomas also presents logical inconsistencies. First, since most patients with recurrent disease initially received high-dose radiation to the area of recurrence, it is not clear why a second treatment should be any more effective than the first. At the very least, one would expect a “narrower” therapeutic window due to the effects of the initial irradiation. Second, an SRS boost as part of the initial treatment of malignant gliomas showed no benefit over conventional radiotherapy alone in RTOG 9305.23 Third, as malignant gliomas have a diffuse, broadly infiltrative component in addition to discrete nodular disease, it is unclear why a strictly “local” treatment of the nodules alone should substantially alter outcome.
Despite these apparent limitations, SRS, HFSRT, brachytherapy, and even conventionally fractionated stereotactic radiotherapy appear to provide reasonable overall survival in comparison to chemotherapy alone for the treatment of recurrent glioma, with median overall survival ranging from 5 to 13 months (typically 8–10 months), as shown in Table 3.2. Note that several of the early studies involving single-fraction SRS reported fairly high rates of late complications (20–40%) requiring reoperation, and that this problem is often viewed as a substantial limitation of SRS.49–52 The use of HFSRT appears to mitigate the rate of adverse radiation events, as shown in the study by Fogh et al.,40 which reported worsened symptoms in only one patient (out of a treatment group of 147 patients) at 6-week follow-up.
Institution | Technique, median dose regimen(s) | Number of patients GBM/total | BVZ at SRS? | Median OS postrecurrence, GBM (months) | Toxicity |
---|---|---|---|---|---|
Minnesota 50 | SRS, 20 Gy × 1 | 26/35 | No | 8 | 31% with reoperation |
Harvard 52 | SRS, 13 Gy × 1 | 86/86 | No | 10 | 48% risk of reoperation @ 2 years |
Minnesota 49 | FSRT, 2.5 Gy × 15SRS, 17 Gy × 1 | 15/2527/46 | No | 7.1 | 30% vs. 8% late complications with SRS vs. FSRT |
Heidelberg 91 | FSRT, 2 Gy × 18 | 59/172 | No | 8 | RN in 1 patient |
Heidelberg 92 | SRS, 15 Gy × 1 | 32/32 | No | 10 | No RN |
Rochester 93 | SRS, 15 Gy × 1 | 18/18 | No | 5.3 | RN in 1 patient |
Jefferson 40 | HFSRT, 3.5 Gy × 10 | 105/147 | Yes (2%) | 10 | 1 grade 3 toxicity |
Sungkyunkwan 51 | SRS, 16 Gy × 1 | 65/114 | No | 13 | RN in 22% |
Henry Ford 64 | SRS, 18 Gy × 1 orHFSRT, 6 Gy × 6 | 26/2610/10 | No Concurrent | 8.47.5 | 2 and 1 patients in SRS and FSRT groups with RN |
Gulhane 94 | SRS, 16 Gy × 1 | 19/19 | No | 9.3 | No grade ≥3 toxicity |
NYU 95 | SRS, 15 Gy × 1 | 16/26 | No | 12.9 | RN in 2 patients |
Haukeland 96 | SRS, 12.2 Gy × 1 | 51/51 | No | 12 | 9.8% complication rate vs. 25.2% in reoperation |
UCSF 97 | SRS, 15 Gy × 1 | 14/26 | No† | 8.8 | Not stated |
Sant’Andrea 98 | HFSRT, 6 Gy × 5 | 38/54 | No‡ | 12.4 (all) | Grade 3 CNS toxicity in 7% |
MSK 43 | HFSRT, 6 Gy × 5 | 20 | Yes (all) | 13 | Grade 3 CNS toxicity in 3 patients |
Duke 42 (retrospective) | SRS, 18 Gy × 1 or 5 Gy × 5 | 49/63 | No (16)Yes (33) | 411 | Crude rate of RN 19% vs. 5% (– vs. + BVZ) |
Henry Ford 62 | SRS, 18 Gy × 1 (7) orHFSRT, 6 Gy ×x 6 | 18/23 | Yes | 7 RT+BVZ vs. 3.3 BVZ alone | Not stated |
Ludwig-Maximilians65 | FSRT, 2 Gy × 18 | 22/30 | No (10)Yes (20) | 5.8 (all)Not reached (all) | One each grade 3 and 4 in BVZ group |
Pittsburgh 61 | SRS, 16 Gy × 1 | 11/11 | No (44§)Yes (11) | 1218 | 9% vs. 46% ARE (+ vs. – BVZ) |
St. Gallen 60 | FSRT, 2.67 Gy × 15 | 8/14 | No (4)Yes (10) | 9 (all) | RN in 1 non-BVZ patient |
Cincinnati 66 | HFSRT, 6 Gy × 5 | 30/35 | Yes (30) | 8.6 (all) | RN in 3 patients (all non-BVZ) |
Duke (prospective) 59 | SRS, 18 Gy × 1 | 8/15 | Yes (all) | 14.4 (all) | 1 grade 3 (headache) |
GliaSite Multinstitutional 99 | I-125 solution, 60 Gy to 1 cm (median) | 95 (80 GBM) | No | 8.3 | 2 patients with grade 3 CNS toxicity (RN), no grade 4 or 5 |
Johns Hopkins 100 | I-125 solution, 45–60 Gy to 0.5–1.0 cm | 24 | No | 9.1 | 2 patients with symptomatic RN, 1 with expressive aphasia |
ARE, adverse radiation event; BVZ, bevacizumab; CNS, central nervous system; FSRT, fractionated stereotactic radiotherapy; HFSRT, hypofractionated stereotactic radiotherapy; GBM, glioblastoma multiforme; OS, overall survival; RN, radionecrosis; RT, radiotherapy; SRS, stereotactic radiosurgery.
Apart from the potential direct antitumor effect of BVZ in the treatment of gliomas, as discussed above, BVZ may offer some specific additional benefits when used in combination with radiotherapy. As described by Moeller et al., 53–55 a paradoxical effect of radiotherapy is the upregulation of hypoxia factor-mediated angiogenesis, an unwanted effect that could be potentially blocked by antiangiogenic agents. In addition, adverse radiation events following SRS appear to be substantially reduced by the use of BVZ.56–58
The combination of BVZ and SRS/HFSRT may provide superior outcome compared to either modality alone.)42,43,59–62 For example, a prospective trial of HSFRT and BVZ from Memorial-Sloan Kettering43 showed that the combination was well tolerated and demonstrated an overall survival of 12 months post-HFSRT. In a follow-up study, the predominant pattern of failure continued to be at or near the site of HFSRT.63 Cabrera et al.59 assessed the toxicity of concurrent BVZ and SRS in a cohort of 15 patients with recurrent malignant gliomas. Only one grade 3 and no grade 4–5 toxicities were observed and quality of life and neurocognition were well preserved following SRS; the median overall survival was 14 months in this group of eight grade IV and seven grade III gliomas.
While the median overall survival with SRS and BVZ appears higher than that of BVZ alone (Table 3.2), this is not a direct comparison of the two approaches. A small retrospective analysis from Henry Ford64 found a substantially higher median overall survival in patients treated with SRS/HFSRT and BVZ than those receiving only BVZ (7 versus 3 months). Several studies have reported low rates of radionecrosis and adverse radiation events in patients treated with SRS/HFSRT and BVZ. For example, in the retrospective study from Duke,42 4/21 (19%) patients treated with SRS alone exhibited symptomatic radionecrosis versus only 2/42 (5%) of the patients receiving both SRS and BVZ. Similarly, in the studies from Memorial-Sloan Kettering,43 Maximilian Ludwig,65 and Cincinnati,66 0, 7, and 9% rates of radionecrosis were observed in patients receiving SRS and BVZ.
Selection bias undoubtedly influences outcome, but the magnitude and direction of that influence in recurrent glioma therapy are unclear. The fact that focal radiotherapy requires a discrete target would exclude those patients with diffuse brain disease from treatment with radiosurgery but not necessarily systemic chemotherapy, for example.
Summary of level 1 evidence
Malignant gliomas
A total of 60 Gy delivered to the partial brain in 1.8–2 Gy daily fractions appears to be the optimal dose regimen. There is no evidence supporting improved outcome from dose elevation via external-beam radiotherapy, brachytherapy, or an SRS boost.
Temozolomide administered concurrently with radiotherapy yields improved overall survival versus radiotherapy alone.
Low-grade gliomas
A total of 50.4–54 Gy to the partial brain in 1.8–2 Gy daily fractions appears to be the optimal dose regimen. There is no evidence supporting the use of higher doses in this disease.
While immediate versus delayed radiotherapy in low-grade glioma increased progression-free survival, there was no difference in overall survival.
In higher-risk low-grade glioma, the addition of PCV to radiotherapy significantly increased progression-free survival and overall survival over radiotherapy alone.
Recurrent gliomas
There is no level 1 evidence on the efficacy of radiotherapy in recurrent gliomas. RTOG 1205 is currently enrolling BVZ-naïve patients with recurrent glioma on a randomized trial of BVZ alone versus HFSRT and BVZ. The targeted accrual for this study is 178 patients with a primary endpoint of overall survival.
Radiotherapy approach and technique
Malignant gliomas
At the time of simulation (typically 2–3 weeks post surgical resection) a custom face mask is fabricated and a high-resolution non-contrast CT scan of the head performed with slice thickness of 1–1.25 mm in the axial plane. In addition, a brain MRI is performed, including T2-weighted, T1-weighted (with and without gadolinium-based contrast) and contrast-enhanced 3D SPGR series. The preoperative and postoperative studies are fused and registered such that the images overlay each other exactly. The preoperative T2-weighted and postoperative contrast-enhancing T1-weighted images (both the resection cavity and residual contrast-enhancing tissue on the latter) are then contoured slice by slice to create gross target volumes.
For malignant gliomas, the primary clinical target volume (CTV primary) is obtained by uniformly expanding the preoperative T2-weighted volume by 2 cm. In the situation where the tumor distorted the normal brain architecture prior to surgery, e.g., producing substantial midline shift, and resection has reduced this distortion, it is also useful to inspect the postoperative T2-weighted volume and appropriately adjust the target volume. As it is essential to adequately irradiate the area at risk, we permit this volume to extend into the posterior fossa and brainstem, though it is removed from the petrous ridge, mastoid process, sella, and orbits. In addition, this volume covers the inner, but not the outer, table of the skull. Using appropriate immobilization and image guidance, this permits adequate dosing of the brain parenchyma at risk, as well as preventing permanent alopecia and, more importantly, wound breakdown and dehiscence. A boost volume (the CTV boost) is then generated by expanding the postoperative contrast-enhanced T1-weighted MR volume (again, which includes the resection cavity) by 1.5 cm, excluding the brainstem, AVPs, and non-target tissues, such as the orbits, sella, and outer table of the skull. In addition, it is valuable to review an immediate postoperative MRI if available, to aid in excluding the intrinsically T1-bright changes secondary to postoperative blood products. In order to meet the dose limitations on the brainstem and AVP, it is usually necessary to permit the CTV boost to come no closer than 2 mm (and preferably 3 mm) to critical structures. Note that we do not add any additional margin to achieve a planning target volume (PTV) for either the primary or boost portions of the treatment plan, as effective immobilization and image guidance render this unnecessary. Thus, the PTV = CTV.
Occasionally, no T2-weighted images are available or no T2-hyperintense volume observed. In this case, the postoperative contrast-enhancing T1-weighted volume plus the resection cavity are expanded 2.5 cm to yield the CTV primary. In the case of the patient who cannot undergo an MRI, we will obtain a head CT with and without contrast during simulation, contour the resection cavity and enhancing tissue, and expand the resulting volume by 2.5 and 1.5 cm to obtain the CTV primary and CTV boost, respectively.
As studies of low-grade gliomas suggest improved retention of neurocognition67 and reduced incidence of radiation-induced optic neuropathy (RION)68 when treating at 1.8 versus ≥2.0 Gy fractions, our preference is to use the lower dose/fraction. Typically, the CTV primary is treated to 45–50.4 Gy (a 5-week course of 1.8 Gy administered each week day). This is immediately followed by treatment of the CTV boost with an additional 14.4–9.0 Gy in 1.8 Gy daily fractions, such that the total prescribed dose is 59.4 Gy.
Because these CTV are often in close proximity to the brainstem and/or AVP, it is usually necessary to employ the 45/14.4 Gy primary/boost prescription in order to limit the dose to these critical structures, even when employing intensity-modulated techniques. The plans are carefully designed and the dose prescribed such that the prescription dose covers 95–98% of the CTV and the maximum dose to the parenchyma is less than 108% of the prescribed dose. When lesions are intimate contact with critical structures, it may be necessary to limit coverage in order to meet dose constraints, even when IMRT is employed. In these cases, our practice is to underdose the boost, administering an adequate dose to all interfaces between the target and critical structures during the primary phase of treatment. These above comments apply to design and selection of doses when we utilize the 46/14 Gy primary/boost regimen (20 × 2 Gy daily fractions to a total of 60 Gy) dictated by many multi-institutional protocols.
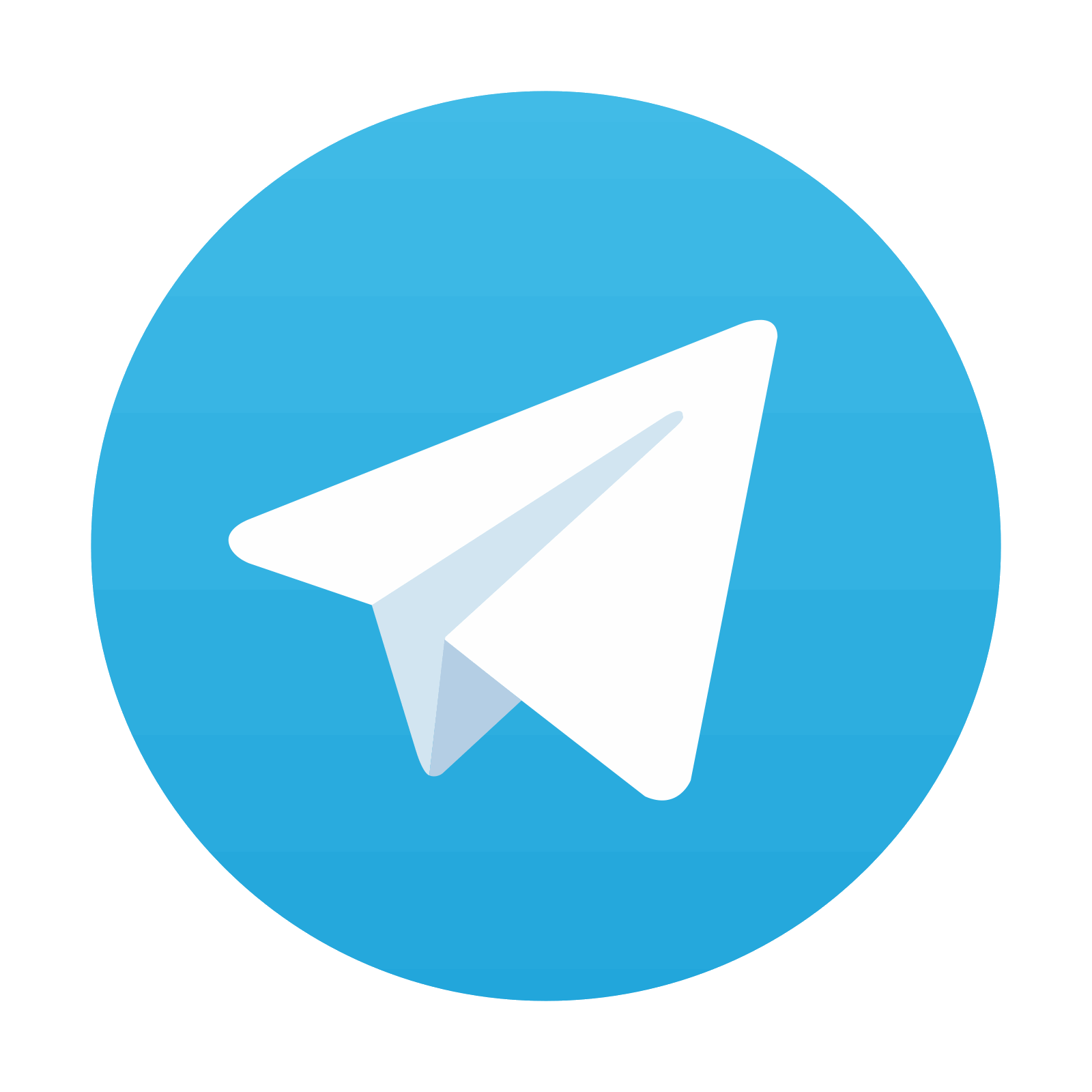
Stay updated, free articles. Join our Telegram channel
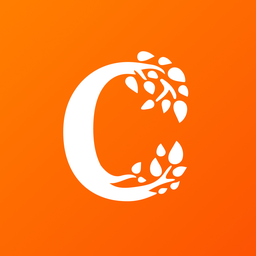
Full access? Get Clinical Tree
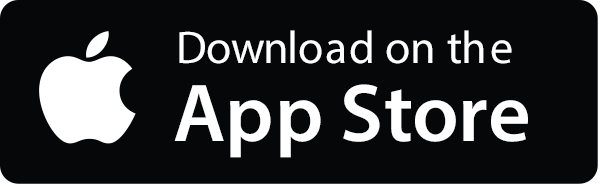
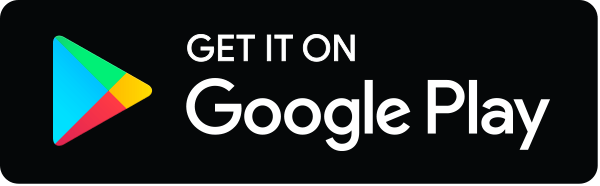