The last few decades have seen great advances in the care of the injured patient from prehospital triage and transport to care in the intensive care unit (ICU). Care in the ICU is designed to reestablish homeostasis and minimize secondary and iatrogenic injury. Excluding early deaths in the operating room, most traumatic hospital deaths will occur in the ICU. More than ever, the outcome of critically injured patients is dependent on a solid understanding of the pathophysiology and evolution of traumatic injuries. Attention to detail is critical and an awareness of the pitfalls is essential if one is to be successful in avoiding preventable morbidity and mortality.
In the last several years, increasing emphasis has been placed on quality of care indicators and physician staffing models for ICUs. A modern surgical ICU in the 21st century provides evidence-based care using algorithms, clinical practice guidelines (CPGs), and checklists; uses cutting-edge technology for physiologic monitoring; and has a robust quality improvement process to continuously evaluate its outcomes and to identify opportunities for improvement.
This chapter focuses on elements of critical care essential to the management of the acutely injured patient, reviews some recent advancements in the monitoring of the critically ill patient, and lists some of the common complications and pitfalls observed in the ICU.
Given the wide variety of clinical expertise and patient populations, several patterns of ICU physician organization have developed. The first is the “closed” unit that relies almost exclusively on a critical care team (or attending intensivist) for primary patient management. Under this scheme, comprehensive management is assumed by the ICU team along with responsibility for all orders and procedures, with other services providing care as consultants on an as-needed basis.
In an alternative model, the “open” unit, there may or may not be a designated ICU director, a separate ICU team, or even an intensivist immediately available to the ICU. Under this system, individual physicians manage and direct intensive care for their respective patients, depending on their institutional privileges. Consultative involvement of a board-certified intensivist is at the discretion of each primary attending physician, and is neither required nor necessarily expected.
Many larger surgical and trauma ICUs have a “semiopen” or collaborative plan of practice whereby the ICU is staffed with intensivists who coordinate care with primary surgeons. While primary surgeons may write orders on their patients, critical care team involvement with each patient is typically either mandatory or expected. There are often specific areas of designated Critical Care autonomy, such as the management of mechanical ventilators, invasive hemodynamic monitoring, pain management, and sedation. In these units, the ultimate responsibility for the patient remains with the primary team, but patient care is a collaborative effort. This semiopen model combines the advantages of critical care expertise for trauma and surgery patients while maintaining primary surgical service responsibility for overall patient management. This arrangement is consistent with Accreditation Council for Graduate Medical Education (ACGME) program requirements for general surgery and neurosurgery training programs as well as guidelines for the optimal care of the injured patient suggested by the American College of Surgeons Committee on Trauma for Level 1 Trauma Centers.1 There is now a growing body of work examining the relationship between ICU staffing models and patient outcomes. For these purposes, rather than trying to compare various models of care, a distinction has been made between “high-intensity” and “low-intensity” physician staffing models. Loosely translated, a “high-intensity” model involves 24/7 dedicated physician staffing and mandatory ICU team involvement with patient management. This includes all closed units and most semiopen units. The remaining “open” units typically utilize “low-intensity” ICU physician staffing. The principal hypothesis is that dedicated, higher-intensity intensivist staffing for ICU patients will ultimately improve outcomes from a variety of conditions and illnesses. In a meta-analysis of 26 pooled studies, Pronovost et al found a relative risk of 0.71 (95% CI = 0.62–0.82) for hospital mortality and 0.61 (95% CI = 0.5–0.75) for ICU mortality associated with high-intensity ICU staffing for adults and children.2 Similar results were found by Nathens et al who specifically examined the effect of high-intensity staffing on outcomes following major trauma.3 Utilizing prospective cohort data from 68 trauma centers, the authors reported a relative risk reduction of 0.78 (95% CI = 0.58–1.04) for ICUs whose patients were either managed (closed unit) or comanaged (semiopen) by board-certified intensivists.
The mortality rate among surgical patients who develop complications, called failure to rescue is also reduced by the presence of intensivists in the ICU. Failure to rescue (FtR) is a quality measure that looks at the hospital mortality rate of surgical patients with complications. Hospitals with intensivists on their rapid response teams or in their ICUs have lower FtR rates.4 The presence of surgical intensivists also reduces complications and improves National Surgical Quality Improvement Program (NSQIP) general surgery quality measures.5
These and other observations have resulted in the creation of ICU staffing guidelines. In 2000, a group of Fortune 500 companies and large public and private health care purchasers formed the Leapfrog Group for the purpose of identifying and creating incentives for sustained patient safety measures in acute care hospitals. This group has identified standards for physician staffing for the ICU that requires the presence of experienced intensivists providing daytime care and response requirements for off-hours care.6
Quality assurance and performance improvement in a surgical ICU are complex processes requiring the ongoing identification of outcome measures or performance indicators, data collection and analysis, and the development of action plans to correct deficiencies and subsequent monitoring of the performance (outcome) measures. The underlying goal of delivering high-quality care also depends on specialization (critical care specialists), provider education and training, good communication and collaborative interaction between specialty and ancillary services. Existing critical care quality assurance programs have used a variety of clinical indicators or “filters” as a measure of the quality of care. These indicators may reflect process measures (eg, percentage of eligible patients receiving deep venous thrombosis prophylaxis in a timely manner), and outcome measures (complication rate [%] for central venous line sepsis). Illness severity indices have been developed for the purpose of predicting outcomes in critically ill patients and are being increasingly used as benchmarking tools, allowing participating units to compare their outcomes with those predicted by the various indices. These performance measurement systems will become increasingly important in allowing managed care organizations to assess program performance. In addition to improved instruments for assessing critical care outcomes, the development and implementation of clinical protocols and CPGs directed at reducing undesirable treatment variability are being linked to ICU performance improvement. Protocols and guidelines, once developed and implemented, can later be analyzed in terms of their clinical efficacy and cost-effectiveness and can be modified further to improve efficiency.
The effectiveness of CPGs has been demonstrated for a variety of problems and conditions including ventilator weaning, pneumonia, nutrition, and sedation.7,8,9,10 The difficulties that most institutions experience, however, relate more to the implementation of CPGs rather than to their development. Current methods of improving implementation of, and compliance with, CPGs include ongoing education, defined order sets, the assignment of some management responsibilities to specialized teams (eg, nutrition and respiratory therapy), and the use of advanced practice staff (nurse practitioners and physician’s assistants).
More recently, several fields in medicine, motivated by an increased awareness of errors in medical care delivery, have incorporated lessons learned from the aviation industry to clinical practice in an attempt to improve quality and patient safety. A checklist is just one of the tools used in the aviation industry that have been tested in many ICUs to potentially improve safety, quality, and consistency of ICU care.11 Inspired by an article by Vincent12 where he described a checklist using the mnemonic FASTHUG (feeding, analgesia, sedation, thromboembolic prevention, head of the bed elevation, stress ulcer prophylaxis, and glucose control), we implemented a modified checklist (mFASTrHUGS) by adding mouth care (m), restraints (r), and skin care (s) (Table 55-1). We use the checklist as a template for daily multidisciplinary rounds and the nursing staff uses it at the end of their shift for turnover. Each item on the checklist is reviewed during rounds assessing its implementation and need. If any item has not been implemented for a particular patient, then the team has to identify a contraindication (eg, pharmacologic DVT prophylaxis during the first day following a significant traumatic brain injury), otherwise the measure has to be implemented. mFASTrHUGS is a package implemented to all patients in our surgical ICU.
Mouth care Feeding Analgesia Sedation Thromboprophylaxis Restraints Head of bed ≥30° Ulcer Glucose Gut Skin care | Teeth cleaning and oral moistening q4h. Commence by day 1 in ICU, follow protocol. Review medication record. Give pain score using verbal, BPS or CPOT. Use PAD algorithm. Review ventilation, GCS, and reason for sedation. Assess RASS score. Daily awakening and sprinting. Prescribe SCDs and/or heparin type or alternative. Assess, reorder, or discontinue restraints. Early mobility and exercise! Foley and lines out? Head of bed up 30° or more. Get C-spine cleared! Review GI ulcer prophylaxis. Maintain optimal glucose control per protocol. Last BM is specified, appropriate bowel protocol. Note potential areas of pressure breakdown; progress to healing. Does patient need special bed? |
One of the characteristics of the ICU is the utilization of intensive physiologic monitoring. Monitoring devices can be used to determine if the patient has stable organ function, and to detect anomalies that may indicate impending organ dysfunction. In recent years there has been a dramatic increase in the ability to monitor a wider variety of physiologic parameters. Monitors can be useful in determining if a patient’s trajectory is deviating from the anticipated or optimal course; however, any monitor’s output requires interpretation and integration with the overall clinical picture. Monitoring itself can be associated with serious and lethal complications, including device related complications and errors in interpretation that lead to errors in clinical decision making. The questions that must be addressed with any form of monitoring include “when?” (indication and timing), “what?” or “how much?” (which specific monitoring tools or techniques), and “why?” (an analysis of the associated risks and benefits).
Hemodynamic monitoring is directed at assessing the results of resuscitation and as a guide to reestablishing and maintaining tissue and organ perfusion (see Chapter 56). The restoration of normal arterial blood pressure, central venous pressure (CVP), pulmonary arterial (PA) pressure, and cardiac output (CO) provides some reassurance that there is adequate organ perfusion. This reduces the likelihood of death and serious morbidity due to complications of hemorrhagic shock including post-injury inflammatory response syndrome and multiple organ failure (see Chapter 61). However, the phenomenon of regional circulation and inadequate perfusion of specific organs, particularly the gut, in the presence of normal arterial pressures remains a concern. New types of monitors are becoming available that can monitor CO and intravascular volume status in a relatively noninvasive fashion, and may be able to determine perfusion at the tissue level.13
Noninvasive blood pressure monitoring is widely available through the use of automated blood pressure cuffs. These devices can be set to perform repetitive blood pressure measurements as frequently as every minute. However, they are usually considered insufficient in patients with significant hypotension due to poor sensitivity at low blood pressures as well as the intermittent nature of the readings. Insertion of an arterial intraluminal catheter or “arterial line” allows instantaneous measurement and continuous display of arterial blood pressure, even at very low blood pressures. Arterial lines have a wide variety of indications (Table 55-2) and are the preferred method of arterial blood pressure management in patients receiving continuous infusions of vasoactive drugs as they allow better drug titration. Arterial lines are typically placed in the radial artery, although they can also be placed in the dorsalis pedis, femoral, or brachial arteries. Infection of arterial lines is less common than that of central venous lines; however, sterile technique should still be utilized to avoid the risk of catheter related blood stream infections. Thrombotic complications can also occur at any arterial line site and may lead to tissue ischemia, including loss of digits. This is particularly common in patients with prolonged hypotension or sepsis or who have been treated with vasopressors. Although Allen tests are frequently performed before the insertion of a radial arterial line, these do not exclude the possibility of this complication.
Arterial line monitoring Shock states (any) Cardiac Septic Neurogenic Hypovolemia/hemorrhage Monitoring intraoperatively/postoperatively Head injury Serial monitoring of ABG Labile hemodynamic status Respiratory failure Hypertensive crisis Vasoactive drug infusions High-risk or elderly trauma patient Central venous pressure monitoring Volume status in acute head injury Complex trauma/pelvic fracture Shock dates Acute renal failure Vasoactive drug infusions Monitoring during acute ICU hemodialysis Cardiac pacing Elderly trauma patient Massive transfusion Pulmonary contusions PA catheter monitoring Refractory septic and cardiogenic shock states Severe hypoxemia, pulmonary edema/embolism/ARDS High-risk intraoperative monitoring Barbiturate coma (iatrogenic myocardial depression) Moderate or mixed venous O2 (Svo2) Diagnoses: explained shock, hypoxemia, renal failure |
Minimally invasive continuous arterial pulse waveform analysis devices are available that quantify pulse pressure variability (PPV), and stroke volume variation (SVV) by analyzing the variations in arterial waveform with respiration and the area under the arterial pressure waveform. These devices can also utilize the calculated stroke volume to provide an estimate of CO without need of a PA catheter. Recent meta-analyses found that PPV and SVV of at least 11–13% both had excellent ability to identify critically ill patients who were likely to respond to fluid challenge with an area under the curve (AUC) of 0.94 and 0.84 respectively.14,15 However, it should be kept in mind the PPV and SVV are most reliable in patients on mechanical ventilation with no spontaneous respirations, and may be less reliable in patients with arrhythmias, spontaneous respirations, as well as those on low tidal volume ventilation.14
Monitoring CVP is most useful in patients at high risk of over or underresuscitation (see Chapter 56). These include patients with limited cardiac reserve, including the elderly and those with cardiac disease. Patients with traumatic brain injury are another group in whom CVP monitoring is frequently performed to avoid hypotension associated with underresuscitation or overresuscitation resulting in increased cerebral edema. Although guidelines suggest CVP goals between 8 and 12 mm Hg,16 these end points may be less reliable in patients on positive pressure ventilation, making trends of CVP over time in response to therapy, rather than static measurements more useful in guiding overall resuscitation. The central venous catheter can also be used to obtain a mixed venous blood gas measurement. Since the mixed venous oxygen saturation (MVo2) is usually slightly lower in the superior vena cava (SVC) as compared with that in the inferior vena cava (IVC) due to the relatively low oxygen consumption (Vo2) of the kidneys, the blood oxygen saturation obtained from a central venous catheter is usually slightly higher than that obtained from a PA catheter. Central venous catheters that have an oximetric tip can measure the saturation of blood at the tip of the central venous catheter or Scvo2, allowing continuous measurement of this marker of global perfusion.
The Swan-Ganz PA catheter was first introduced in 1970; however, in the mid-1990s there were a number of studies questioning their efficacy and reports of complications including pulmonary infarction and pulmonary artery thrombosis, perforation, bleeding, and pseudoaneurysm. More recent studies have not demonstrated increased major adverse sequelae associated with the PA catheter.17 However, these studies also failed to demonstrate any significant improvement in outcomes associated with PA catheters. Currently PA catheters are primarily utilized in patients who have known or suspected myocardial dysfunction.
An alternative to invasive PA catheter placement is the FloTrac/Vigileo (Edwards Lifesciences, Irvine CA, USA) system which utilizes arterial waveform analysis to calculate SVV, stroke volume, and CO. Initial studies of this technology were disappointing,13 but recent meta-analyses suggests the technology has improved with better concordance with PA catheter and echocardiographic estimates of CO with a percentage of error less than or equal to 30%.15 However, this optimal concordance only occurs in patients with normal ejection fraction (EF ≥40%) and SVR.15,18,19 Other minimally invasive devices utilize arterial waveform analysis in conjunction with lithium dilution (LiDCO [Cambridge, UK]), and thermodilution (PiCCO [PULSION medical system, Munich Germany]) to estimate SVV and CO. However, these devices require placement of a central venous catheter, calibration every 8 hours, and are also less accurate among patients with arrhythmias, valvular disease, depressed cardiac function and high or low SVR.19
The formal transthoracic echocardiogram (TTE) can be invaluable in assessing the function and structure of the heart as well as the health of its components including valves and chambers. Limited TTE can also detect such conditions as pneumothorax and pleural effusions.18,20 However, in about 50% of trauma patients it is difficult or impossible to perform a complete TTE due to chest trauma, subcutaneous air, obesity, or surgical dressings. Transesophageal echocardiography (TEE) can overcome many of these limitations and can be used in the detection of such injuries as blunt aortic rupture (see Chapter 26).13,18,19,20 However, frequent use of TEE in the ICU can be limited due to availability, cleaning requirements, and the skill required by the operator. However, there are now FDA-approved TEE devices using a disposable probe that can be left in the esophagus or stomach for up to 72 hours allowing for repeated exams over time. Studies of this catheter demonstrate excellent image quality and utility in directing therapeutic decision making.13,19,21
Transthoracic impedance, also known as bioimpedance cardiography, uses an electrical current applied to the thorax. Changes in bioimpedance to this current are used to measure heart rate, left ventricular EF, and intrathoracic fluid content and calculate stroke volume, CO, and SVR. However, the technology is limited by patient movement, and arrhythmias; and is inaccurate in pregnancy, obesity, pleural fluid or gas, heart failure, severe valvular disease, and pulmonary edema. A new device is available that utilizes impedance electrodes on the outside surface of an endotracheal tube, Endotracheal Cardiac Output Monitor (ECOM, ConMed, Irvine, CA). This allows impedance measurements without interference from abnormalities or motion of the chest wall. However studies have demonstrated an inability to accurately estimate or trend CO when compared to thermodilution.13,22
Near-infrared spectroscopy (NIRS) is a noninvasive technique in which a probe utilizing near-infrared light is applied to the thenar eminence. This allows detection of the tissue oxygen saturation (Sto2) of the muscle of the thenar eminence. Decreased Sto2 correlates with increased mortality and organ failure in patients with hemorrhagic shock or sepsis.23
Blood transfusions are common in the ICU with 30–62% of critically ill patients receiving an average of 2–4 U of packed red blood cells.24,25 Blood transfusions independent of shock or injury/disease severity are associated with worse outcomes. Increased infection, multiple organ dysfunction, and mortality are directly correlated with the amount of blood transfused.25,26 Lowering target hemoglobin from 10–12 to 7–9 g/L was associated with improved outcomes in ICU patients in the Transfusion Requirements in Critical Care (TRiCC) study.27 The TRiCC trial results have been reaffirmed by multiple subsequent studies and meta-analyses in various ICU and surgical populations including cardiac disease, respiratory failure, and those on mechanical ventilation. In addition to improved survival these studies also found that restrictive transfusion strategies were associated with reductions in rebleeding, acute coronary syndrome, pulmonary edema, and bacterial infections without adversely affecting functional recovery or length of stay.28,29 Despite initial support for liberal transfusion among septic patients, subsequent studies have failed to demonstrate any benefit to transfusion triggers above 7 g/L within this population.27,30 The updated Surviving Sepsis Campaign Guidelines from 2012, support a transfusion trigger of less than 7.0 g/dL with a target hemoglobin concentration of 7–9 g/dL.16 The appropriate trigger for patients with traumatic brain injury remains unclear. However, recent studies have demonstrated increased mortality with transfusions in traumatic brain injury patients with hemoglobin levels greater than 10 g/dL, no benefit in neurologic outcome, and a significantly increased risk of thromboembolic events and transfusions with a trigger of 10 g/dL when compared to a trigger of 7 g/dL.31,32
A general approach to transfusion is that patients with hemorrhage need transfusion, while those who are not bleeding infrequently require immediate transfusion. In the trauma bay, operating room, and ICU, red blood cells are often the most available and effective initial resuscitation fluid, and prudence suggests a liberal transfusion strategy until hemorrhage control is achieved.
One source of anemia well within the control of the surgical intensivist is phlebotomy related blood loss. A study in 2012 demonstrated average ICU phlebotomy losses to be 436 mL for all comers and 679 mL for patients with an ICU length of stay of at least 4 days.33 Studies have also demonstrated small changes in the amount of blood drawn, as little as 3.5 mL/d, can significantly increase risk for blood transfusion.24 Concerted efforts should be made in the ICU to reduce the number of serial labs, eliminate unnecessary studies, and utilize minimal or pediatric tubes for all necessary tests. Additionally, technology such as continuous noninvasive hemoglobin monitors may avoid unnecessary serial blood tests, and have been shown to reduce the percent of patients receiving transfusions as well as the average volume of blood transfused per patient.26
The anemia of critical illness resembles that of chronic inflammatory disease; low circulating erythropoietin levels are found in critical illness and are thought to be one of the causative factors. Although epoetin alfa and darbepoetin alfa have been widely accepted for anemia due to chronic kidney disease, these agents are also attractive for anemia of critical illness as they may restore hemoglobin levels without transfusion. However, the optimal hemoglobin targets and dosing have not been established and clinical trials (EPO-1 and EPO-2) demonstrating improved hemoglobin levels or transfusion reduction used target hemoglobin higher than those suggested by TRiCC.34,35 Subsequent studies (EPO-3) while demonstrating an increase in hemoglobin with Erythropoiesis-stimulating agent (ESA) failed to find a reduction in transfusions.36 A subset analysis of the trauma patients in the EPO-2 and EPO-3 studies suggested there was approximately a 50% reduction in 29-day mortality with ESAs.37 This survival benefit was redemonstrated in patients with traumatic brain injury.38 However, there is no clear consensus on ESA dosing in trauma patients, and increased adverse outcomes, including micro and macrovascular thromboembolic events and increased mortality have been seen in ESA trials utilizing high dose regimens and higher hemoglobin targets.39 Optimal response to ESAs may not be achieved in the presence of relative iron deficiency, which is common in ICU patients. Concomitant iron administration has been recommended with ESAs, but randomized, clinical studies have not shown improved transfusion rates with enteral iron supplementation.40
One of the principal indications for admission to the ICU for trauma patients is the need for frequent neurologic assessments in those with known or suspected traumatic brain injury (see Chapter 19). There are two goals for monitoring traumatic brain injury: first, the avoidance of secondary brain injury by avoidance of hypoxia and hypotension and maintenance of cerebral perfusion; second, the detection of increased intracranial pressure (ICP) due to cerebral edema or expanding intracranial hematomas. Reassessment should be done frequently as even subtle changes may herald increases in ICP or cerebral ischemia.
ICP monitoring with a ventriculostomy catheter or subdural bolt is a common practice in most major centers for patients who have a neurologic exam that is unavailable or unreliable following traumatic brain injury. The Brain Trauma Foundation (BTF) guidelines recommend early and aggressive monitoring of ICP and the calculated cerebral perfusion pressure (CPP), which is the difference between the mean arterial pressure (MAP) and ICP.41 Monitoring of the ICP and CPP can be used to guide therapy such as vasopressors, sedation, paralysis, hyperosmolar therapy, or cerebrospinal fluid drainage and to provide surveillance for increasing cerebral edema or intracranial hemorrhage. Adherence to BTF guidelines, including compliance with placement of ICP monitors has been associated with improved mortality in patients with traumatic brain injury.42,43,44
Investigational noninvasive monitoring systems for ICP include transocular ultrasound monitoring of optic nerve sheath diameter (ONSD).45 The optic nerve sheath is an extension of the intracranial dura matter and has been shown to become distended with elevated ICP. Continuous transcranial Doppler ultrasound, brain tissue oxygen, and cerebral microdialysis monitoring are other investigational approaches for ICU monitoring of traumatic brain injury that may contribute to decision making and eventually improve outcomes in these patients.46
Current guidelines indicate the need for nutritional assessment and monitoring for patients admitted to the ICU (see Chapter 60). Enteral nutrition, which may help preserve gut mucosal barrier function, is the preferred approach. Nutritional assessment should begin with an assessment of the degree of pre-injury malnutrition as well as current requirements. Nutritional monitoring in the ICU is controversial but can include calculation of nitrogen balance, weight, prealbumin, and albumin. The literature to date suggests serial weight measurements and serum albumin predict outcomes such as mortality and length of stay poorly in the critically ill. In comparison, nitrogen balance (goal 2–4 g positive nitrogen balance) and prealbumin more accurately predict outcomes.47 Indirect calorimetry (metabolic cart) provides an assessment of caloric requirements and metabolic rate. The device calculates Vo2 and carbon dioxide production (Vco2). Standard metabolic carts requiring an integral mechanical ventilator were intended for intermittent use; however, newer devices can be used with standard ventilators with an adapter that can then continuously sample end-tidal oxygen and carbon dioxide. Co2 and Pco2 can be used to then determine the respiratory quotient (RQ) and caloric needs can be estimated. The RQ can also be used to determine the prominent nutritional substrate being used, excess carbohydrates leading to RQs of 1.0 or more, with RQs below 0.8 indicating possible excess lipid utilization.
Hyperglycemia with or without insulin resistance appears to be a common phenomenon in critically ill patients. Studies have also shown that hyperglycemia in trauma patients correlates with higher mortality rates.48 The van den Berghe trial demonstrated that tight glucose control (at or below 110 mg/dL) with intensive insulin therapy improved mortality and reduced complications such as infection rate, multiorgan failure rate, ventilator days, and morbidity.49 However, the intensive insulin regimen initially proposed by Van den Berghe et al is associated with increased hypoglycemia and has failed to show a mortality benefit in subsequent multi-institutional studies such as Glucontrol and Normoglycemia in Intensive Care Evaluation Survival Using Glucose Algorithm Regulation (NICE-SUGAR).50,51 In the NICE-SUGAR trial, 90-day mortality was actually increased in patients assigned to intensive insulin therapy, as compared with an intermediate target range for blood glucose. Improved glucose control is still a guideline used in most ICUs; however, a moderate protocol (goal glucose level <180 mg/dL) rather than an aggressive one is most likely to result in optimal prevention of hyperglycemia without inducing higher mortality or hypoglycemic events.16,48
The normal response to physiologic stress is to increase levels of tissue corticosteroids; this is also seen in response to critical illness. Failure to recognize and treat adrenal insufficiency has been associated with increased mortality. Normal corticosteroid response can be impaired in a variety of conditions including sepsis, systemic inflammatory response syndrome (SIRS), and traumatic brain injury. Identification of patients with acute adrenal insufficiency can be difficult; random cortisol sampling (<15 mcg/dL) and corticotropin stimulation tests (15–34 mg/dL) have been used in the past but are not sensitive or specific. Patients with abnormal stimulation tests (increases <9 mg/dL) were felt to require corticosteroid supplementation. While a 2002 study by Annane et al suggested a benefit to low-dose steroids in sepsis, the 2008 multi-institutional CORTICUS trial did not show any 28-day mortality benefit with 300 mg/d of hydrocortisone in patients with septic shock, either overall or in patients who did not have a response to corticotropin.52,53 However, hydrocortisone did hasten reversal of shock in CORTICUS study patients. Lacking adequately powered positive studies, low-dose steroid therapy (200 mg/d) should be limited to patients with septic shock whose blood pressure is poorly responsive to fluid resuscitation and vasopressor therapy.16 Corticotropin stimulation has no role in determining steroid use in patients with septic shock.16 Steroids should be discontinued if the patient does not respond to treatment given the potential risks of infection, hyperglycemia, and critical illness polyneuropathy.
Mortality after trauma in the ICU is often attributed to infection. Infections are thought to contribute to more than 88,000 ICU deaths annually in the United States.54 Infectious complications of major injury (see Chapter 18) may be due to the results of the injury itself (eg, open fractures), as a result of complications of treatment (eg, anastomotic leak), or as iatrogenic complications of critical care management (eg, ventilator-associated pneumonia [VAP] and central line infection). Specific monitoring for infections will depend on injury type and severity, types of interventions performed, and the duration of post-injury critical illness. Regulatory authorities have recently mandated surveillance cultures on admission to the ICU for certain health care–associated infections (HAIs) such as nasal swabs for methicillin-resistant Staphylococcus aureus (MRSA). In a study using multiple regression analysis, the most common predictors of infection were central venous catheters, mechanical ventilation, chest tubes, and trauma with open fractures.55 Patients at risk for infectious sequelae should be routinely tested by culture and examined for clinical indications such as fever, leukocytosis, change in physical examination, pyuria, and development of purulent sputum or new infiltrate on chest x-ray. HAIs such as central line–associated bloodstream infection (CLABSI) may have standardized definitions in some jurisdictions and regulatory requirements for surveillance. Other HAIs such as VAP may require adoption of a local or institutional standard definition and therapy given the lack of a national consensus. The decision to start presumptive (empirical) antibiotics should be based on risk factors, the expected sequelae of injury, and prior infections. Presumptive treatment should be started, if indicated, stopped at a defined end point for culture-negative patients, and appropriately deescalated when final culture information and sensitivities are available. Importantly, not all critically injured trauma patients with unexplained fevers require antibiotics. Fever in these patients may have a noninfectious origin (Table 55-3) or be due to occult infection that has not been considered, successfully cultured, or has no identifiable signs or symptoms. Fungal sepsis should be considered in patients with prolonged ICU stays, multiple prior antibiotic therapies, and immunosuppression (see Chapter 18).
Rhabdomyolysis SIRS/sepsis syndrome Adrenal cortical insufficiency Pheochromocytoma (rare) Drug fevers Transfusion reaction Malignant hyperthermia Malignant neuroleptic syndrome Pancreatitis Burn injury Pulmonary embolism/DVT Alcohol and drug withdrawal CNS hemorrhage |
The need for mechanical ventilation is the most common indication for admission to the ICU. The general principles for intubation and mechanical ventilation are the following:
Secure and establish an airway.
Decrease the work of breathing (WOB).
Improve oxygenation.
Improve ventilation (CO2 gas exchange) and maintain control of Paco2 especially in acute brain injury.
Anticipate worsening respiratory status or airway patency such as the need for large-volume resuscitation, severe neck/thoracic trauma, upper torso/facial burns, and inhalation injury.
Current ventilator technology is diverse and advanced; however, the essential physical principle of mechanical ventilation is pushing oxygen-rich air into the lungs by positive pressure and removal of waste CO2 by reduced pressure. Ventilator management can change rapidly; therefore, a nucleus of critical care expertise is needed not only to optimize respiratory support but also to interpret acute changes in pulmonary mechanics that can often be a harbinger of systemic pathology.
Rapid assessment of arterial oxygen tension (Pao2) is essential for both evaluating and managing the adequacy of alveolar–arterial oxygen gas exchange. Oxygen (O2) is driven from the alveolar airspace into the pulmonary capillaries along a diffusion gradient between the respective tissue beds. Calculating the efficiency of pulmonary oxygen exchange can be cumbersome since the equation for the A–a gradient requires alveolar and arterial CO2 concentrations, shunt fraction, water vapor pressure, and body temperature. A more convenient and simple bedside index of oxygen exchange is the Pao2/Fio2 (P/F) ratio that adjusts for a fluctuating Fio2 and helps in defining lung injury. The use of pulse oximetry to determine arterial oxygen saturation (Sao2) has replaced continuous arterial blood gas measurements as a real-time, noninvasive method to assess arterial oxygenation. There are limitations to Sao2 and its nuances are important in interpreting the significance of an absolute Sao2 percentage. First, because of the kinetics of oxygen–hemoglobin binding, Sao2 and the oxygen dissociation curve is sigmoidal and not linear. Therefore, small changes in Sao2 may reflect a much larger drop in Pao2. Second, under instances of carbon monoxide poisoning or severe circulatory shock, oxygen delivery will be abnormally low despite a near-normal Sao2.
In addition to oxygenation adequate ventilation, end tidal (Et)CO2, and resultant arterial CO2 tension (Pco2) have important physiologic and clinical implications and must be monitored in mechanically ventilated patients. The immediate detection of CO2 through qualitative capnography relies on the lower pH of EtCO2-rich air changing the color of pH-sensitive filter paper in the capnograph. As a rule, a disposable capnograph remains purple if EtCO2 is less than 0.5% and turns yellow when EtCO2 is greater than 2.0%.56 Normal EtCO2 is greater than 4%; therefore, capnography turns yellow when the endotracheal tube is positioned in an airway. This device is very sensitive unless the patient is in circulatory arrest and adequate pulmonary perfusion is compromised. The presence of a large volume of acidic gastric contents may give a false impression of successful endotracheal intubation in some cases of esophageal intubation. In these circumstances, initial detection of EtCO2 decreases rapidly with subsequent tidal volumes. Continuous quantitative capnography analyzes EtCO2 and, depending on the alveolar–arterial gradient, can give an indication of the arterial Pco2. In patients without preexisting pulmonary disease, the normal alveolar–arterial gradient is between 1 and 3 mm Hg. Utilizing quantitative capnography can be helpful in brain injury where hyperventilation may curtail rising ICP. However, EtCO2 measurements are affected by pulmonary dead space fraction and pulmonary perfusion that can be greatly altered in cases of hemorrhagic shock, thoracic trauma, and increased airway resistance. Warner et al performed a prospective study of 180 freshly intubated trauma patients and correlated EtCO2 measurements with Pco2 from blood gas analysis.57 Using regression analysis, the authors found a direct correlation between EtCO2 and Pco2; however, patients ventilated with an EtCO2 of 35–40 mm Hg were likely to have a Pco2 greater than 40 mm Hg 80% of the time, and a Pco2 greater than 50 mm Hg 30% of the time. In severely injured patients, where an increase in pulmonary shunt may exist, the measurement of Pco2 through blood gas analysis remains the most accurate measure of assessing ventilation.
Causes of hypoventilation can be multifactorial. Increased dead space from pulmonary contusion, acute lung injury (ALI)/acute respiratory distress syndrome (ARDS), pulmonary embolism, and oversedation can be contributing factors causing hypercapnia especially during the weaning phase of mechanical ventilation.
Assessing pulmonary mechanics can significantly aid in diagnosing and treating a patient’s sudden or progressive pulmonary insufficiency. One of the most important parameters to measure is compliance and the ability to distinguish between static and dynamic compliance. Compliance, the change in volume produced by a change in pressure, is calculated based on measurements taken from the ventilatory circuit itself. In general, a sudden decrease in compliance will mean a concomitant rise in both plateau pressure (PP) and peak inspiratory pressures (PIPs) for a given tidal volume (VT) as seen in pneumothorax or abdominal compartment syndrome. However, measuring the individual changes of either static or dynamic compliance may indicate a specific pulmonary pathology.
In static compliance, the change of volume produced is measured from the inspiratory hold pressures or plateau pressure using the following formula: Compliancestatic = VT/PP – PEEP. Static compliance reflects the alveoli and chest wall, rather than the airways. Cases of decreased static compliance (normal = 50–100 mL/cm H2O) with normal or elevated PIP generally indicate intra-alveolar pathology such as ARDS, ALI, and pulmonary edema.
Dynamic compliance utilizes the PIP (Compliancedynamic = VT /PIP – PEEP) to estimate resistance of the airways. Sudden decreases in dynamic compliance or a measured difference between static and dynamic compliance reflect increased airway resistance during bronchospasm, mucous plugging, kinked endotracheal tube, or foreign body aspiration.
A well-accepted principle in mechanical ventilation is the importance of increasing alveolar recruitment to improve oxygenation. Assessment of the pressure–volume curve in mechanical ventilation has been utilized to determine the mean pressure needed for the inflection point, or Pflex (Fig. 55-1). This point represents the critical pressure needed to open collapsed alveoli and corresponds to the zone of optimal alveolar recruitment. The pressure reading at the inflection point may reflect an optimal PEEP setting. In ARDS, ALI, and pulmonary edema the normal pressure–volume curve is shifted (Fig. 55-1) leading to derecruitment of alveolar units and increasing pulmonary shunt. During these instances, increasing driving pressure and PEEP are often employed to maximize gas exchange.
Ventilator capacity encompasses the ability of a patient to generate muscular forces needed to meet the ventilatory work required for physiologic demand. Assessing ventilatory capacity in the mechanically ventilated patient can be an important physiologic indicator of clinical improvement and predict successful extubation, particularly in patients who have been intubated for prolonged periods of time, or have neurologic (spinal cord injury and myasthenia gravis) or mechanical (flail chest and rib fractures) injury affecting the thorax. Forced vital capacity (FVC) and negative inspiratory force (NIF) are the most frequently measured indices of ventilator capacity. A normal vital capacity is between 65 and 75 cm3/kg depending on ideal body weight and sex. The NIF estimates inspiratory muscle strength and a value of –25 to –30 cm H2O is indicative of adequate inspiratory force to maintain airflow after extubation.58
Spontaneous breathing utilizes negative intrathoracic pressure to fill alveoli (Fig. 55-2). In contrast, mechanical ventilation utilizes positive pressure to fill alveoli and negative pressure for exhalation. Positive pressure mechanical ventilation can improve gas exchange by recruiting atelectatic alveoli, increasing functional residual capacity (FRC), and reducing areas of ventilation/perfusion mismatch, thereby decreasing pulmonary shunt fraction. Negative effects of mechanical ventilation vary according to ventilatory mode; however, adverse effects common to all positive pressure modes include barotrauma, ventilator-induced lung injury, and impairment of cardiac output from decreased venous return. It is important to assess the physical mechanics behind each mode of ventilation since correct management of ventilated patients will decrease total ventilation days and improve patient outcomes. In choosing which mode of ventilation is best suited for a particular patient, it is helpful to evaluate the patient’s current oxygenation, ventilation, pulmonary compliance, muscular strength, and mental status.
Both continuous mandatory ventilation (CMV) and assist-control (AC) mode ventilation are preset volume-cycle modes that deliver a fixed tidal volume at a specific respiratory frequency. The individual names of these modes are commonly referenced interchangeably; however, CMV delivers a set tidal volume exclusive of a patient’s ventilatory effort, whereas AC synchronizes delivery of a set tidal volume to each patient-initiated breath. Consequently, an awake and breathing patient will have episodes of respiratory dysynchrony causing significant discomfort, breath stacking, or barotrauma.59 Because of this CMV is rarely used.
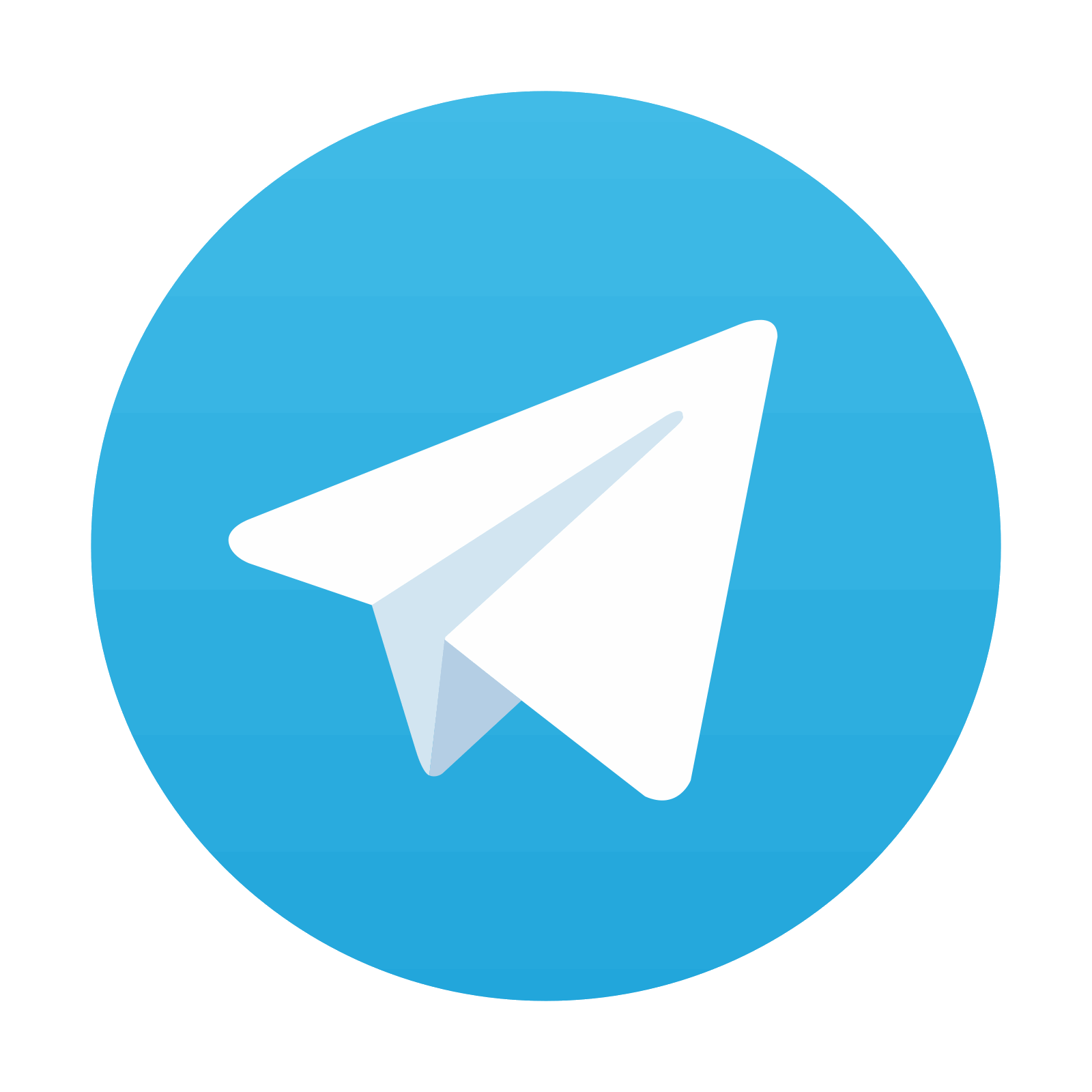
Stay updated, free articles. Join our Telegram channel
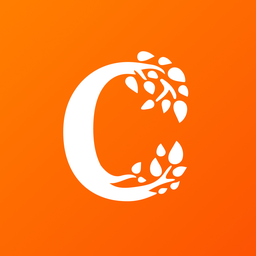
Full access? Get Clinical Tree
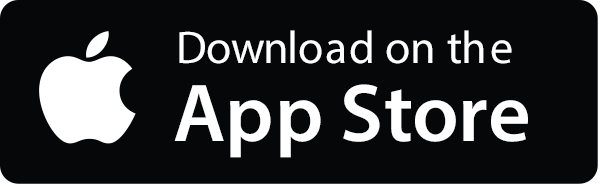
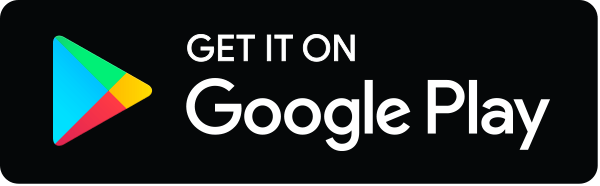