The purpose of this article is to provide a review of innovations in radiation oncology that have been recently adopted as well as those that are likely to be adopted in the near future. Physics and engineering innovations, including image-guidance technologies and charged particle therapy, are discussed. Biologic innovations, including novel radiation sensitizers, functional imaging for use in treatment planning, and altered fractionation, are also discussed.
Key points
- •
Innovations in image guidance have made radiation delivery more accurate and reproducible and may reduce side effects and improve effectiveness.
- •
Charged particle therapy, such as proton radiation, can overcome the physical limitations of X rays but has some practical limitations.
- •
Sensitizers are commonly used during radiation to improve tumor control, and protectors are being developed to reduce radiation’s adverse effects.
- •
Functional imaging, such as positron emission tomography, is already used to define targets of radiation and may eventually be used to design more intelligent and complex treatments.
- •
Altered fractionation is becoming less alternative because many fractionation schemes such as those used in radiosurgery are being studied and adopted.
Introduction
The practice of radiation oncology has changed greatly in the past few decades and continues to change at a brisk pace. These changes are fed by innovations in engineering, computing, physics, and biology.
An example will help to illustrate the changes that have taken place in radiation oncology. Let us compare a sample patient with rectal cancer treated today with one treated several decades ago. A few decades ago, radiation oncology was practiced in 2 dimensions (ie, with radiographs instead of computed tomography [CT]). A radiation oncologist would have obtained plain radiographs of this patient’s pelvis and would have drawn fields by hand with crayon on the radiographs similar to the one shown in Fig. 1 . The design of these fields would have been based on an understanding of soft tissue anatomy and the natural spread of disease, but bony anatomy would have served as the only direct guide. A technician would have cut blocks comprised of a lead-based alloy to match these drawings, and these heavy blocks would have been placed in the machine head daily by radiation therapists at the time of treatment. Radiation doses would have been calculated by hand to a few points of interest.
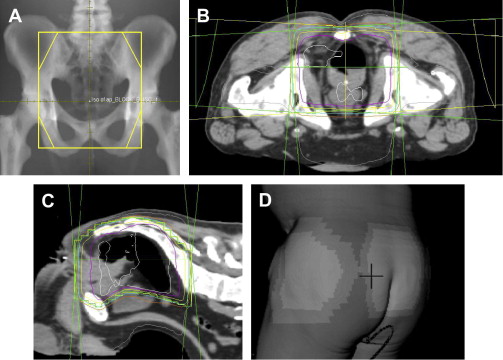
Today, nearly all patients undergoing radiotherapy are planned using 3-dimensional information from a CT. The radiation oncologist sits in front of a computer and draws fields with a mouse that can be viewed in any dimension. Fig. 1 B–D provides examples of some of the information available to radiation oncologists when planning a treatment. Inclusion of a target or exclusion of normal tissues is achieved with more confidence. Doses to targets and normal structures (in this case femoral heads, bladder, small bowel, and so forth) can be accurately and quickly determined to any point in 3 dimensions. What ensues is an iterative process whereby the radiation oncologist fine-tunes a plan in an environment in which the consequences of field changes are immediately calculated and known. Furthermore, treatment-planning software itself is capable of some decision making in a process known as inverse planning .
The delivery of radiotherapy has also changed. Field-shaping blocks are rarely cut by hand because treatment machines are now equipped with small movable leaves that are able to shape fields quickly and easily. Checking the patients’ position during radiation has also improved substantially. Once limited to the use of plain radiographs, radiation oncologists may now choose from a wide variety of image-guidance techniques that provide 3-dimensional information and can, in some situations, even monitor patients’ position while the beam is on. Finally, patients today commonly undergo treatment with chemotherapy concurrent with a course of radiotherapy.
This review describes innovations in radiation oncology as well as exciting, potentially field-changing technologies still in development. The authors describe the practical implications as well as the limitations of these innovations. The authors have attempted to include the most influential and recent innovations, but this report is in no way exhaustive; because of space constraints, the authors have excluded concepts that some would deem quite important.
Introduction
The practice of radiation oncology has changed greatly in the past few decades and continues to change at a brisk pace. These changes are fed by innovations in engineering, computing, physics, and biology.
An example will help to illustrate the changes that have taken place in radiation oncology. Let us compare a sample patient with rectal cancer treated today with one treated several decades ago. A few decades ago, radiation oncology was practiced in 2 dimensions (ie, with radiographs instead of computed tomography [CT]). A radiation oncologist would have obtained plain radiographs of this patient’s pelvis and would have drawn fields by hand with crayon on the radiographs similar to the one shown in Fig. 1 . The design of these fields would have been based on an understanding of soft tissue anatomy and the natural spread of disease, but bony anatomy would have served as the only direct guide. A technician would have cut blocks comprised of a lead-based alloy to match these drawings, and these heavy blocks would have been placed in the machine head daily by radiation therapists at the time of treatment. Radiation doses would have been calculated by hand to a few points of interest.
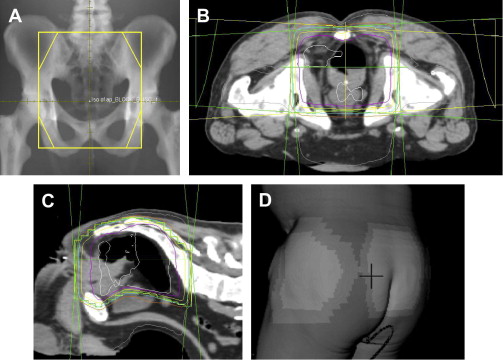
Today, nearly all patients undergoing radiotherapy are planned using 3-dimensional information from a CT. The radiation oncologist sits in front of a computer and draws fields with a mouse that can be viewed in any dimension. Fig. 1 B–D provides examples of some of the information available to radiation oncologists when planning a treatment. Inclusion of a target or exclusion of normal tissues is achieved with more confidence. Doses to targets and normal structures (in this case femoral heads, bladder, small bowel, and so forth) can be accurately and quickly determined to any point in 3 dimensions. What ensues is an iterative process whereby the radiation oncologist fine-tunes a plan in an environment in which the consequences of field changes are immediately calculated and known. Furthermore, treatment-planning software itself is capable of some decision making in a process known as inverse planning .
The delivery of radiotherapy has also changed. Field-shaping blocks are rarely cut by hand because treatment machines are now equipped with small movable leaves that are able to shape fields quickly and easily. Checking the patients’ position during radiation has also improved substantially. Once limited to the use of plain radiographs, radiation oncologists may now choose from a wide variety of image-guidance techniques that provide 3-dimensional information and can, in some situations, even monitor patients’ position while the beam is on. Finally, patients today commonly undergo treatment with chemotherapy concurrent with a course of radiotherapy.
This review describes innovations in radiation oncology as well as exciting, potentially field-changing technologies still in development. The authors describe the practical implications as well as the limitations of these innovations. The authors have attempted to include the most influential and recent innovations, but this report is in no way exhaustive; because of space constraints, the authors have excluded concepts that some would deem quite important.
Image guidance
Introduction
A typical daily radiation treatment lasts at least several minutes. If a patient’s position deviates from what is expected even by a small amount, the tumor may be underdosed or normal tissue may be inappropriately exposed to radiation. Dealing with patients’ position and motion is one of the fundamental issues of radiotherapy. If there is no mechanism in place to check the patients’ position, a treatment machine has no knowledge of the patients’ location and the linear accelerator will continue ignorantly streaming X rays regardless of where the patients may be. To reduce the chance of motion error, almost all modern radiotherapy is guided by imaging obtained in the treatment room with patients in the treatment position.
The simplest, and most frequently used, form of image guidance involves acquiring plain radiographs while patients are in the treatment position. These portal films are simple to acquire and are adequate for many situations, but there are significant limitations. First, soft tissues (which are often the most relevant for localizing tumors) are poorly visualized. Second, image quality in general is often poor because of the use of a very high-energy photon beam optimized for treatment rather than imaging ( Fig. 2 ). Third, portal films acquired before treatment yield no information about motion while the radiation beam is on.
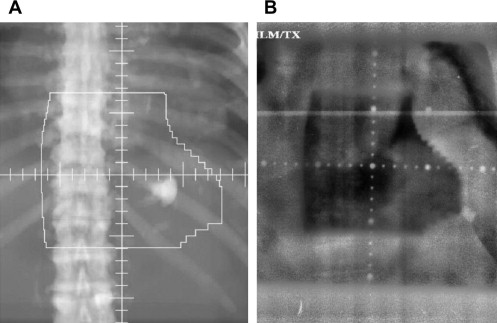
The limitations of using portal films for image guidance are well demonstrated with the example of prostate cancer treatment. Although the bony pelvis may seem to be positioned properly, the prostate may move substantially between or even during radiation treatments. For example, a rectal air bubble may displace the prostate anteriorly over 1 cm. A treatment plan using portal imaging alone for image guidance would be ignorant of this motion. Faced with this knowledge, the treating radiation oncologist is faced with either expanding the margins of the radiation fields, which will place more normal tissue in the field, or using narrow fields but risk missing the target. In modern radiation oncology, more advanced forms of image-guided radiotherapy (IGRT) are commonly used for situations in which portal films are inadequate. Most of these technologies have become common only in the past decade. (It is important to note that IGRT typically refers to technologies more advanced than portal films, although literally, radiographs are a form of image guidance.)
IGRT for Interfraction Motion
Interfraction motion refers to changes in patient position from one treatment to the next. A course of radiation typically consists of many individual treatments (or fractions) of radiation delivered daily. There are many methods to account and correct for interfraction motion that are superior to portal films. One solution is to obtain 3-dimensional imaging with the use of CT. A CT scan may be acquired in the treatment vault in several manners. First, a treatment vault may contain a CT scanner that is literally built on rails and is able to roll over the treatment table and the stationary patient ( Fig. 3 ). Second, some treatment machines have the capability of generating a CT by rotating the gantry 360° around patients while acquiring an image; the result of this technique is termed a cone beam CT . There are many other ways of accounting for interfraction motion, such as through the use of ultrasound or by implantation of radiopaque markers in soft tissues that can be visualized easily with films. Although very useful for many situations, the main limitation to all of these methods is that they do not account for motion during treatment or intrafraction motion. If patients move during treatment, radiation continues unperturbed.
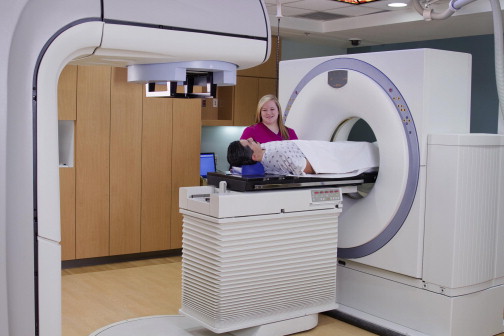
IGRT for Intrafraction Motion
There are many systems that can account for intrafraction motion. These systems can automatically stop treatment or sound a warning if movement is detected and will resume once adjustments are made. Let us again use the example of prostate cancer. One solution to intrafraction motion for prostate cancer uses radiofrequency transponders that are implanted into the prostate before the start of radiation. During radiation, sensors track the position of these transponders, which act as a surrogate for prostate position. If one of the transponders moves outside of its expected range, treatment stops, and the radiation therapists reposition the patient. This radiofrequency transponder method can localize the prostate to within a few millimeters.
Intrafraction motion is particularly relevant in cases when tumors are in or adjacent to the lung and move with respiration. The traditional and simple method of compensating for respiratory motion is to expand the borders of the radiation fields to achieve adequate margins throughout respiration, with the obvious drawback being larger fields with increased dose to normal tissues. More advanced solutions to respiratory motion management include systems that predict the position of the target and turn the beam on and off as the target moves into and out of position in a process known as respiratory gating . Respiratory gating systems do not directly see the tumor. Instead, these respiratory gating systems track an easily detectable surrogate marker on the skin or implanted near the tumor and predict the position of the target based on these surrogates.
For example, the authors’ institution uses a stereoscopic system, which is a collection of cameras that can recognize body position. This system essentially watches the rise and fall of the abdomen and from this information can predict where the target is throughout the breathing cycle. Respiratory gating is often used in the treatment of breast cancer, for which radiation fields may include significant cardiac tissue at full expiration, but greatly exclude the heart during inspiration. For the treatment of breast cancer, patients are often instructed to inhale and hold their breath to increase the distance between the chest wall and heart.
A drawback of respiratory gating systems is that the radiation beam is turned on and off with each breath and the total duration of treatment is increased. There is at least one commercially available system, the CyberKnife (Accuray, Sunnyvale, CA), that actually moves with the target throughout respiration. The CyberKnife is a compact linear accelerator that is mounted on a powerful robotic arm that is capable of very fine and fast movements ( Fig. 4 ). The x-ray beam remains on as the robotic arm moves back and forth with respiration, keeping the beam on target. There are other respiratory tracking systems in development that use a robotic couch that moves patients with each breath instead of moving the beam.
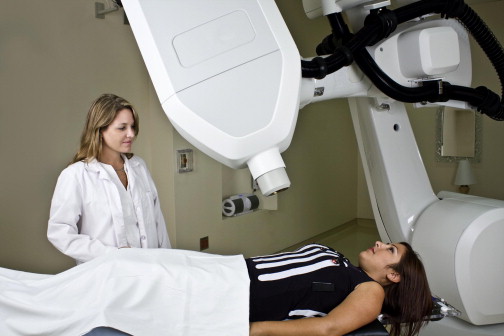
The Future of Image Guidance
The ideal image-guidance system would track real anatomy instead of surrogate markers, continuously track during treatment, and make automatic adjustments to compensate for movement. To this end, radiotherapy machines coupled with magnetic resonance imaging (MRI) are in development by at least 2 organizations, one based in Germany and another in Ohio. These machines are being designed to continuously and quickly acquire MRI images during radiotherapy and to automatically interpret images within small fractions of a second. Automatic and fast interpretation of images is a substantial computational challenge. Successful development of MRI-coupled linear accelerators, therefore, would represent both an engineering and computing achievement. If and when these devices are used in patient care, they have the potential to be a very important tool for IGRT.
Particle beam therapy (protons and so forth)
The Problem with X Rays
Despite all of the innovative gadgets adopted by radiation oncologists in the past decades, they are still limited by the physical laws of high-energy X rays, which are used to deliver most radiotherapy. An x-ray beam loses a constant percentage of its energy per distance traveled through a patient. A typical x-ray beam used for treatment loses about 3% of its dose per centimeter traveled. The x-ray beam’s remaining energy decreases exponentially through a patient and the beam exits on the other side of the patient, destined toward the wall of the vault with substantial remaining energy. The usual solution is to aim multiple x-rays beams from many angles at the target, so that only at the convergence of the beams is the dose high (as a rough approximation of this procedure, think of 4 flashlights aimed at a single spot). Even with these efforts, there is substantial dose deposited in tissues where it is unwanted.
Charged Particle Radiation, in Theory
High-energy protons and other charged particles behave in a fundamentally different way. They deposit little dose through much of their path, then deposit more dose at the end of their paths, resulting in a pattern termed a Bragg peak ( Fig. 5 ). Furthermore, the depth of the Bragg peak can be adjusted either by changing the energy of the proton or by adding material between the proton source and the body. With these methods, a tumor may be treated to a high dose while sparing shallower normal tissues. Because protons save much of their dose deposition for the end of their paths, a treatment with protons will typically deliver less dose to normal tissues than will a photon treatment.
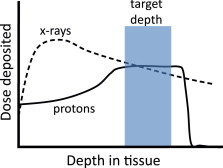
Studies have evaluated the theoretical advantage of protons over X rays. Studies comparing protons with X rays for prostate cancer show that protons reduce mean rectal dose 25% to 59% and mean bladder dose 19% to 50%. Similarly, proton therapy for lung cancer reduces the dose to the lung, spinal cord, esophagus, and heart. Perhaps the largest theoretical benefit in using proton therapy is in the treatment of pediatric patients requiring radiotherapy to the brain or the craniospinal axis. Normal tissues of children and adolescents likely have higher inherent radiosensitivity, and children also have a lifetime to experience late effects of treatment. Proton therapy for craniospinal irradiation for pediatric medulloblastoma has been explored and demonstrates an impressive reduction in the radiation dose to normal tissues. An illustration of this concept is in Fig. 6 .
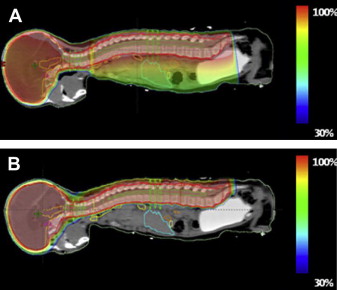
Carbon ions offer further theoretical advantages over protons. Carbon ions, like protons, deposit more energy at the end of their paths in the form of a Bragg peak. Unlike X rays or protons, carbon ions have a characteristic termed high relative biologic effectiveness (RBE). RBE is a measure of how effective radiation is per unit of energy deposited. Simply put, particles with a high RBE, such as carbon ions, kill more cells for a given amount of energy deposited. Furthermore, for carbon ions, the RBE increases only at the end of the path, whereas in the earlier portions of the path, the RBE is the same as X rays or protons. Practically, this property of carbon ions means that not only is the dose delivered where it is wanted but the dose also has more effect than that of X rays or protons. Carbon ions have another potential advantage. As they deposit dose in tissue, carbon ions generate positron-emitting isotopes. If a patient is placed in a positron emission tomography (PET) scanner immediately following treatment, the dose deposited may be directly evaluated. Although this application may seem obscure, the ability to directly visualize and confirm dose deposition is quite exciting to the radiation oncologist.
Charged Particle Radiation, in Practice
Radiation oncologists are drawn toward particle therapy because of these theoretical advantages and the attractive plans generated by their treatment planning systems, but the actual clinical benefits of particle therapy are not so easily known, and the literature lacks good randomized evidence in its favor. Furthermore, there are potential disadvantages to proton therapy when compared with x-ray therapy. First, because of the exquisite sensitivity of the path of protons to the patients’ position and tissue type, they are less forgiving than X rays and there is more uncertainty as to where radiation is actually being delivered. To compensate for this uncertainty, the region targeted with radiation is typically slightly larger, which might place normal tissues at a higher risk of adverse effects. Second, image-guidance and field-shaping technology lags behind x-ray radiotherapy, although this problem may be overcome given more time and engineering advancements.
The decision to treat with particle therapy is often based on a predicted reduction in normal tissue toxicity, but this improvement is often predicted to be small. For example, for the treatment of prostate cancer with intensity modulated radiation therapy (IMRT), the modern rate of grade 3 late genitourinary and gastrointestinal toxicities may be as low as 5% and 1%, respectively. These data suggest little room for improvement for these parameters beyond current x-ray–based therapy. In addition, the irradiated volumes that result in toxicity are often in the target so that they will always receive a high dose of radiation regardless of the modality. A recent retrospective study has shown no detectable reduction in morbidity with protons for prostate cancer, possibly because of the limitations described in the previous paragraph.
Cost is certainly one of the single most significant limitations to the widespread adoption of particle therapy. Individual proton facilities cost between $100 million and $225 million. Even with some very favorable assumptions, some individuals have argued that the cost is not worth the potential small gains for many cancers. Despite these astronomic costs and the practical limitations of protons, there is a proton boom in the United States. Based on the Particle Therapy Co-operative Group’s Web site, which tracks all facilities treating with particle therapy, there are currently 34 proton and 6 carbon ion operating facilities in the world. The United States has 10 operating proton facilities, with 6 more under construction. When the dust settles, proton and carbon ion therapy will likely have an important place in the treatment of certain cancers, but particle therapy seems unlikely to replace X rays as the standard modality for most treatments any time soon.
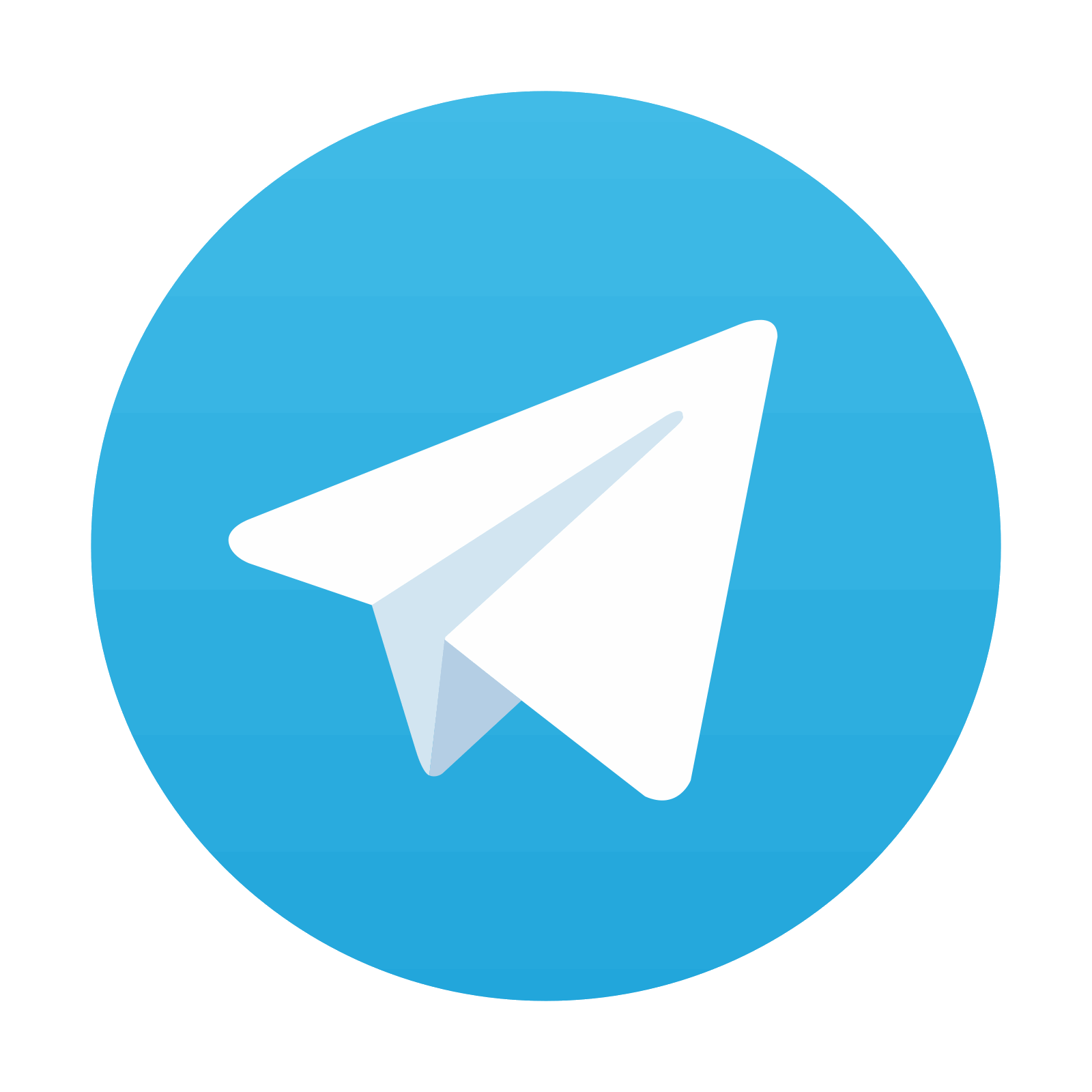
Stay updated, free articles. Join our Telegram channel
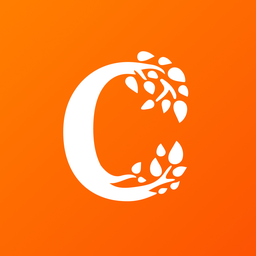
Full access? Get Clinical Tree
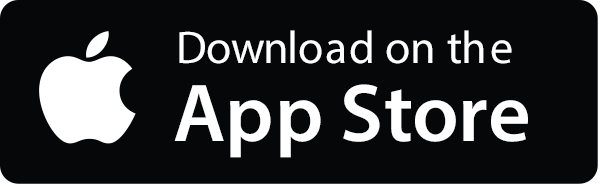
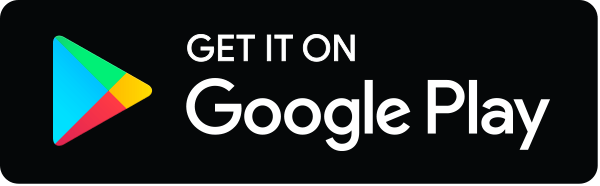