Fig. 14.1
BDNF signaling impacts biological processes throughout the human body. BDNF binds to the TrkB receptor, resulting in receptor dimerization and autophosphorylation. Binding to the TrkB receptor activates multiple downstream pathways, including PLCγ, PI3K/AKt, and Mapk/Erk. Through PLCγ, BDNF increases intracellular calcium concentration and activates calcium-binding molecules such as CaMKIIα, leading to CREB activation and regulation of gene transcription. BDNF-dependent Erk activation also phosphorylates CREB. Both Erk and mTOR regulate translation by binding to the cellular translational machinery. Through these pathways, BDNF regulates cell function, growth, and survival. In the brain, BDNF increases dendritic arborization (Horch and Katz 2002; Jaworski et al. 2005; Cheung et al. 2007; Takemoto-Kimura et al. 2007; Tanaka et al. 2008; Je et al. 2009; Lazo et al. 2013) and spine growth and number (Tyler and Pozzo-Miller 2001, 2003; Alonso et al. 2004) in neurons (1). Synaptic strengthening through long-term potentiation has been shown to require BDNF (2) (Korte et al. 1995; Kang et al. 1997; Rex et al. 2007). BDNF is also required for the formation and maintenance of memory (3) (Tyler et al. 2002; Heldt et al. 2007; Pardon 2010; Bekinschtein et al. 2007). Mechanisms underlying the effects of BDNF in the brain have been an active area of study, and critical roles for post-transcriptional regulation by BDNF have been demonstrated in the biological processes illustrated in boxes 1–3. BDNF-dependent modulation of dopaminergic and serotinergic networks regulates mood, anxiety, and depression (4) [see reviews by Martinowich and Lu (2007) and Nikulina et al. (2014)]. BDNF regulates energy intake and body weight through its signaling in numerous hypothalamic nuclei [see review by Rios (2013)], and modulates stress response through the hypothalamic-pituitary axis (Givalois et al. 2001; Givalois et al. 2004; Jeanneteau et al. 2012 (5). Outside of the central nervous system, BDNF expression is low under basal conditions in mature bone cells and is upregulated in osteoblasts responding to bone fracture (6) (Kilian et al. 2014). BDNF also is known to promote the development and survival of B cells (7) in the immune system (Schuhmann et al. 2005; Fauchais et al. 2008). BDNF expression in the ovary has been shown to regulate follicular development and oocyte maturation (8) (Paredes et al. 2004; Spears et al. 2003; Jensen and Johnson 2001). In the vasculature, BDNF plays a role in the maintenance of blood vasculature and pericyte function (9) (Donovan et al. 2000; Anastasia et al. 2014). As novel functions of BDNF are discovered in multiple biological systems, our knowledge of the post-transcriptional mechanisms contributing to BDNF action is expected to continue to expand
The subcellular localization and release sites of BDNF in CNS neurons has been investigated in multiple reports, but studies have often been hampered by the low levels of endogenous BDNF message and protein in vivo and in vitro, making detection difficult. Bdnf mRNA has been reported in both the neuronal soma and dendrites depending on the length of its 3′ UTR . However, recent research using deep-sequencing and high-resolution in-situ hybridization approaches indicates that all forms of bdnf mRNA appear to be restricted to the soma and proximal dendrites (Will et al. 2013). Similarly, BDNF has been observed in the synaptic compartment and was thought to be released from both axonal and dendritic sites, while recent research indicates that endogenous BDNF is primarily stored in pre-synaptic vesicles (Andreska et al. 2014). Conflicting data regarding the subcellular location and release sites of BDNF protein and message may in part arise from discrepancies between the behavior of endogenous BDNF and the exogenous BDNF expression constructs sometimes employed. Additionally, post-endoplasmic reticulum trafficking of secretory cargo, presumably including BDNF, has been shown to be subject to both developmental and acute spatial regulation in dendrites, where synaptic activity acts to restrict long-range transport of cargo (Hanus et al. 2014).
BDNF interacts with the high affinity tropomyosin-related kinase B (TrkB) tyrosine kinase receptors to activate multiple intracellular signaling cascades, including the MAPK, mTOR, and PLCγ pathways. Signaling downstream of TrkB receptors mediates the trophic effects of BDNF on neurons and synaptic connections, and blocking the BDNF/TrkB interaction results in significant impairments in synaptic plasticity and learning (Takei et al. 2001; Tanaka et al. 2008; Lai et al. 2012). Enduring effects of BDNF on growth and plasticity require changes in gene expression, and BDNF has been shown to regulate both transcription and translation. The precise complement of proteins present in neurons and at synapses fundamentally shapes neuronal growth and activity, ultimately determining synaptic response. Through the activation of select transcription factors such as serum response factor (SRF), nuclear factor kappa B (NF-κB), and CREB (cAMP response element binding protein, Finkbeiner et al. 1997; Kalita et al. 2006; Riccio et al. 2006; Kajiya et al. 2009), BDNF is able to specifically upregulate trophic targets at the level of transcription. However, recent high-throughput approaches have shown that the cellular transcriptomes appear to correlate only moderately with corresponding proteomes (Tian et al. 2004; Schwanhausser et al. 2011; Ideker et al. 2013), revealing that the levels of many proteins are predominantly controlled post-transcriptionally. These studies collectively highlight the importance of understanding mechanisms of post-transcriptional regulation of gene expression. Given the dramatic role that BDNF plays in neuronal health, survival, and plasticity, understanding how BDNF signaling can generate trophic programs of gene expression at a post-transcriptional level has the potential to reveal critical regulatory points in both normal and abnormal brain function, and will be the central theme of this chapter.
2 BDNF Regulation of Global Protein Synthesis
Activity-dependent alterations in neuronal protein synthesis have long been known to be required for the endurance of synaptic changes and memory consolidation (Pfeiffer and Huber 2006; Gal-Ben-Ari et al. 2012). For example, secretion of BDNF during neuronal activity regulates translation, which allows for modulation of the neuronal proteome and accounts in part for the important role of BDNF in synaptic plasticity and cognition (Klann and Denver 2004; Soule et al. 2006; Santos et al. 2010). BDNF signaling through its TrkB receptor increases global cellular protein synthesis as measured by metabolic label incorporation in rodent cortical or hippocampal cultured neurons (Takei et al. 2001, 2009; Huang et al. 2012). The role of BDNF in upregulating total translation has also been demonstrated in studies using brain slices from transgenic mice engineered to either lack or overexpress the gene encoding BDNF. Brain slices from BDNF-deficient mice exhibit a significant decrease in basal translation, whereas basal translation is increased in brain slices from transgenic mice that overexpress BDNF (Takei et al. 2009).
The three major steps of eukaryotic translation involve translation initiation, elongation, and termination, and BDNF has been shown to enhance total cellular protein synthesis by influencing molecular components of both the initiation and elongation phases. In eukaryotic cells, translation is believed to be primarily regulated at the initiation phase, which begins when eukaryotic initiation factor 4E (eIF4E) binds to the 7-methyl-guanosine residue that constitutes the 5′ cap of the mRNA. eIF4E binding to the mRNA allows recruitment of additional initiation factors, ultimately leading to ribosomal association with the eIF-mRNA complex. In the mammalian brain, eIF4E itself is regulated by eIF4E-binding protein 2 (4EBP2), which binds to and negatively regulates eIF4E (Banko et al. 2005). BDNF signaling through TrkB results in phosphorylation of 4EBP by the PI3K/mTOR pathway, which causes 4EBP to dissociate from eIF4E, leading to increased eIF4E activity and generally enhancing mRNA translation. Additionally, eIF4E itself may be phosphorylated by BDNF-mediated activation of the MAPK pathway, which appears to lead to stabilization of eIF4E and, again, enhanced total translation (Gingras et al. 1999, 2004; Takei et al. 2001; Kanhema et al. 2006). BDNF also decreases phosphorylation of eukaryotic initiation factor 2a (eIF2a) and increases phosphorylation of its guanine exchange factor eIF2B, both of which have been shown to enhance binding of tRNA to the ribosome (Takei et al. 2001).
BDNF acts on the elongation step of translation primarily by regulating the activity of eukaryotic elongation factor 2 (eEF2 ). eEF2 is a GTP-binding protein that is responsible for translocation of the tRNA from the A to P site in the ribosome, and is therefore a crucial regulator of the rate of elongation. Following treatment with BDNF, the translation elongation rate in neurons has been reported to be twice that of baseline, as measured by ribosomal transit time (Inamura et al. 2005). Phosphorylation of eEF2 is inactivating and thought to arrest translation, although translation of some mRNAs may be selectively upregulated when eEF2 is phosphorylated (Scheetz et al. 2000). BDNF appears to increase total levels of eEF2 protein (Takei et al. 2009), while decreasing phospho-eEF2 in an mTOR-dependent manner (Inamura et al. 2005; Takei et al. 2009). In contrast, infusion of BDNF into the dentate gyrus of adult rats lead to a rapid, transient increase in eEF2 phosphorylation in whole cell homogenates but not synaptodendrosomes (a subcellular fraction containing a portion of the dendritic shaft and associated spines; Kanhema et al. 2006). Such results suggest that while BDNF-mediated control of translation initiation may be widespread, control via translation elongation could be more compartment or transcript-specific. Collectively, these alterations in both initiation and elongation contribute to the modest BNDF-mediated upregulation of protein synthesis observed by global measures such as radiolabel incorporation.
3 BDNF Regulates the Local Protein Composition in Dendrites and Synapses
Neurons are structurally complex cells with elaborate dendritic and axonal processes that extend significant distances from the cell soma. Both passive and active mechanisms can mediate transport of proteins and mRNAs to distal processes. However, following exposure to an appropriate incoming stimulus, the levels of selective proteins are also specifically and rapidly upregulated at post-synaptic sites, as a component of synaptic plasticity . The finding of both mRNAs and polyribosomes in dendrites and dendritic spines, the major site of excitatory synaptic input, provided the initial suggestion of a role for local translation in dendrites and synapses (Steward and Levy 1982; Steward and Schuman 2001; Ostroff et al. 2002). Activity-dependent local translation is now well-established and is known to be required for multiple forms of plasticity (Sutton and Schuman 2006; Wang et al. 2009; Holt and Schuman 2013). A number of studies have been aimed at compiling a profile of the synaptic transcriptome, with over 2500 transcripts reported to be localized to the synapse via deep sequencing (Cajigas et al. 2012). As might be expected, a number of mRNAs that code for proteins involved in such processes as neuronal signaling, synaptic plasticity, and synapse organization are preferentially enriched in synaptic and dendritic compartments (Poon et al. 2006; Zhang et al. 2006; Cajigas et al. 2012). The mRNAs for some important plasticity-related proteins, such as CamKIIα, were reported to be present, although not enriched, in the dendritic cytoplasm, suggesting the possibility that preferential local translation of these mRNAs could contribute to the abundance of the proteins at synapses (Poon et al. 2006; Zhong et al. 2006).
Mechanisms through which BDNF increases global protein synthesis, as previously discussed, can also function to increase protein synthesis in dendrites and synapses (Takei et al. 2004; Kanhema et al. 2006). For example, BDNF leads to phosphorylation of 4EPB1 in both the dendrite and synapse in an mTOR–dependent manner (Takei et al. 2004; Kanhema et al. 2006). Additionally, in isolated synapses, BDNF causes the inhibitory protein CYFIP1 to dissociate from eIF4E, leading to enhanced translation (Napoli et al. 2008). Similarly, phosphorylation of p70S6K via BDNF activation of mTOR results in phosphorylation of ribosomal S6 protein, increasing translation activation at the synapse (Takei et al. 2004). In other cell types, it is thought that the association of ribosomal subunits, translation factors, and mRNAs with F-actin functions to hold the molecular players in an ordered state and facilitate translation. BDNF has been reported to cause the translocation of eIF4E into spines where it associates with cytoskeleton-bound RNA granules, an effect that is blocked by inhibiting F-actin polymerization (Smart et al. 2003).
Not surprisingly, neuronal studies on local protein regulation by BDNF have often focused on the upregulation by BDNF of proteins that are known to play important roles in synaptic function and plasticity. Increased translation of multiple specific mRNAs such as glutamate receptor subunits, Homer2, and CamKIIα is observed at synapses in response to BDNF (Schratt et al. 2004; Caldeira et al. 2007; Guire et al. 2008; Fortin et al. 2012). In a study performed by Aakalu et al. (2001), the authors constructed a GFP reporter containing the 5′ and 3′ UTRs of CamKIIα, to reveal that stimulation with BDNF led to translational “hot spots” in dendrites that may correlate with active synapses. BDNF has also been shown to increase the levels of certain mRNAs in dendrites (Ying et al. 2002; Rao et al. 2006; Messaoudi et al. 2007), supporting the function of BDNF as a regulator of local gene expression. It is worth noting that BDNF has also been implicated in modulating synaptic protein content through post-translational modifications. For example, BDNF-induced phosphorylation of both NDMA and AMPA receptor subunits, leads to increased surface trafficking and activity of these receptors (Suen et al. 1997; Lin et al. 1998; Caldeira et al. 2007). Evidence also suggests that BDNF may post-translationally regulate synaptic protein stability through the ubiquitin proteasome pathway (Jia et al. 2008), and that neuronal activity leads to increased proteasomes in the synapse, which can function to remodel synaptic protein content (Bingol and Schuman 2006).
4 Effect of BDNF on Translational Specificity
4.1 BDNF Increases Translation of a Select Group of mRNAs
Long-term changes in synaptic and circuit function are known to require changes in gene expression. Enduring strengthening or weakening of synapses each necessitate organized alterations in programs of gene expression in order to ensure that the appropriate proteins are coordinately regulated to enhance or reduce synaptic responses. BDNF effectively promotes synapse growth and plasticity by coordinating a response that specifically and selectively increases plasticity-related proteins. Despite the ability of BDNF to affect general translational machinery and total cellular translation, the modest increase in global translation that is observed in response to BDNF has actually been attributed to a robust effect on a relatively small number of specific transcripts. Using a candidate-based approach, several initial studies demonstrated that BDNF can increase the translation of specific plasticity-related proteins—such as CamKIIα, Arc, and glutamate receptor subunits—in both dissociated neuronal culture and synaptic preparations (Aakalu et al. 2001; Yin et al. 2002; Kelleher et al. 2004; Takei et al. 2004; Kanhema et al. 2006; Jourdi et al. 2009). In contrast, BDNF has been observed to decrease translation of certain mRNAs, such as potassium channels and co-transporters (Rivera et al. 2002; Raab-Graham et al. 2006). Radiolabelled synapses stimulated with BDNF and subjected to 2D electrophoresis also revealed a robust increase in a very specific set of proteins, while some proteins were decreased and the majority were unchanged (Yin et al. 2002).
The development of high-throughput techniques enabled an appreciation of the truly impressive extent to which BDNF mediates target specificity. In a comprehensive study from the Greenberg lab (Schratt et al. 2004), the authors used polysome profiling to show that only roughly 4 % of transcripts present in neurons were increased by BDNF stimulation, and that these increases were sensitive to mTOR signaling. Additionally, multidimensional protein identification technology (MudPIT) demonstrated that a brief, 30 min BDNF stimulation of isolated synapses was sufficient to specifically increase proteins involved in synaptic vesicle formation and trafficking, translation, and synaptic components (Liao et al. 2007). In line with previous studies, the translation from highly selective pools of mRNAs were specifically increased as well as decreased by BDNF, while most mRNAs remained unchanged (Liao et al. 2007). Similarly, gel based proteome profiling revealed that a long-term (12 h) BDNF stimulation could increase levels of a number of proteins involved in cellular metabolism and proliferation, while most protein levels were decreased or unchanged (Manadas et al. 2009). Interestingly, the authors reported that BDNF was capable of affecting both the mRNA and protein levels of its targets, but that these changes were not always correlated, again implicating post-transcriptional roles for BDNF in regulating de novo protein synthesis or protein stability (Manadas et al. 2009).
4.2 Mechanisms Enabling BDNF -Mediated Translational Specificity
The ability of BDNF to rapidly and significantly increase the translation of a restricted subset of mRNAs is in accordance with its role as a positive modulator of growth and excitatory synaptic function, but how is BDNF able to confer such a remarkable degree of specificity to translational processes?
4.2.1 RNA-Binding Proteins
One well-established method of achieving translational specificity is through RNA-binding proteins, which play important roles in post-transcriptional regulation of mRNAs by enhancing or repressing the translation of certain mRNAs. Several different RNA-binding proteins have been observed both to be present at synapses , and to regulate the translation of major targets of BDNF (Huang et al. 2002; Napoli et al. 2008; Wu et al. 1998; Bhakar et al. 2012). For example, the RNA-binding protein CPEB (cytoplasmic polyadenylation element-binding protein) regulates mRNA translation by mediating cytoplasmic polyadenylation. CPEB binds to a cytoplasmic polyadenylation element (CPE) present in the 3′ UTR of multiple BDNF target mRNAs, such as CamKIIα (Huang et al. 2002; Wu et al. 1998). CPEB also associates with an inhibitory eIF4E binding protein , Maskin , which when present in the CPEB/Maskin/eIF4E complex specifically prevents translation of bound CPE-containing mRNAs. Activity-dependent phosphorylation of CPEB causes increased cytoplasmic polyadenylation, which disrupts the Maskin/eIF4E interaction, leading to increased translation of CPE-containing mRNAs (Richter and Sonenberg 2005). In this regulatory pathway, CPEB phosphorylation in response to synaptic activity has been shown to result in an accumulation of CamKIIα at the synapse (Huang et al. 2002). Although CPEB regulation has not been directly studied in relation to BDNF-mediated post-transcriptional gene target specificity, the fact that BDNF initiates several major phosphorylation cascades and increases translation of many CPE-containing mRNAs suggests that BDNF could potentially regulate cytoplasmic polyadenylation in the dendrite and synapse.
As discussed, BDNF regulates translation both locally and globally through mTOR-dependent phosphorylation of 4EBP2, leading to enhanced eIF4E-dependent initiation. Though this pathway might initially be expected to non-selectively increase translation of mRNAs, recent research has shown that 4EBP2 knockout does not significantly alter the neuronal polysome profile, but instead leads to increased translation of specific mRNAs such as neuroligins (Gkogkas et al. 2013). In addition, several studies have demonstrated a specific requirement for 4EBP2-dependent modulation of eIF4E activity in LTP and memory. These results indicate an important role for such molecules in translation regulation and might suggest selectivity for proteins involved in synaptic plasticity (Banko et al. 2005; Costa-Mattioli et al. 2009). Ultimately these investigations imply that 4EBP2-mediated regulation of cap-dependent initiation could contribute to translational specificity for a subset of transcripts in response to BDNF rather than mediating a general increase in translation.
BDNF signaling has also been linked to another RNA-binding protein, the Fragile X Mental Retardation Protein (FMRP ), an RNA-binding protein that is enriched at CNS synapses and could participate in conferring specificity to BDNF-mediated translation. Loss of FMRP results in human Fragile X Syndrome, which is an autism spectrum disorder and the most common monogenic inheritable form of intellectual disability. FMRP is generally thought to function as a repressor of protein synthesis, but participates in the regulation of translation through multiple incompletely characterized mechanisms (Bhakar et al. 2012). Loss of FMRP leads to exaggerated translation of a variety of synaptic proteins including many major targets of BDNF, such as CamKIIα, Arc, Map1B, and APP (Napoli et al. 2008; Bhakar et al. 2012). FMRP function and expression has been directly linked to BDNF signaling in multiple studies (Castren et al. 2002; Napoli et al. 2008; Wang et al. 2012b; De Rubeis et al. 2013). BDNF decreased FMR1 mRNA levels in cultured hippocampal neurons, and both FMR1 mRNA and FMRP protein levels were found to be decreased relative to controls in transgenic mice overexpressing the BDNF receptor TrKB (Castren et al. 2002). The phosphorylation status of FMRP may play a role in its inhibition of translation, and dephosphorylation of FMRP has been suggested to enhance translation of FMRP target mRNAs (Ceman et al. 2003; Narayanan et al. 2007). One study directly examining the relationship of BDNF to FMRP phosphorylation showed that BDNF caused calcineurin-mediated dephosphorylation of FMRP, leading to increased translation of FMRP targeted mRNAs (Wang et al. 2012b). Similarly, PP2A, another phosphatase that is reported to dephosphorylate FMRP (Niere et al. 2012), is itself increased by BDNF (Takei et al. 2009). Research aimed at understanding the mechanism by which FMRP regulates translation has suggested that FMRP may work in part by binding to the protein CYPIP1 to harbor FMRP mRNA targets in the FMRP-CYPIP1 complex. CYPIP1 itself also binds to and sequesters eIF4E, preventing translation of these targets (Napoli et al. 2008; De Rubeis et al. 2013). BDNF was shown to decrease co-immunoprecipitation of CYPIP1 and eIF4E, suggesting that eIF4E is released and able to initiate translation of FMRP target mRNAs (Napoli et al. 2008; De Rubeis et al. 2013).
4.2.2 microRNAs
Although the discovery of microRNA -mediated regulation of protein synthesis is relatively recent (Lee et al. 1993), in the past 20 years many ground-breaking studies have demonstrated the importance of microRNA (miRNA) function in all cell types. miRNAs are short (19–25 nucleotide), non-coding RNA molecules that are endogenously expressed. They are able to recognize and bind partially-complementary sites in their target mRNAs, causing translational suppression and/or degradation of these targets. miRNA function in the brain has been shown to be crucial for normal neuronal development and plasticity in both mammalian and non-mammalian species (Krichevsky et al. 2003; Sempere et al. 2004; Giraldez et al. 2005; Smalheiser and Lugli 2009) Given that a single miRNA can regulate expression of an entire suite of proteins, miRNAs are attractive candidates for coordinating complex responses such as neuronal development, plasticity and synaptic remodeling at a post-transcriptional level. There is strong evidence for the presence of both miRNAs and miRNA processing machinery at the synapse (Schratt et al. 2006; Ashraf et al. 2006; Wayman et al. 2008; Huang et al. 2012; Lugli et al. 2012), further supporting a potential role for miRNAs in synaptic plasticity . The miRNA processing enzyme Dicer has been found to be present and active in synaptosomes, and there is additional evidence for enrichment of both precursor (pre-miRNAs) and mature miRNAs at synapses (Lugli et al. 2005, 2008). Interestingly, enrichment of mature miRNAs has been correlated with the presence of the corresponding pre-miRNA, again suggesting that miRNA processing might happen locally at the synapse (Lugli et al. 2008). Though it is still not known how miRNAs could be directed or trafficked to a particular synapse, it has been suggested that synaptic pre-miRNAs might be enriched for pre-miRNA structures with shorter stems and more bulges, offering a potential structural basis for their transport as opposed to immediate processing (Smalheiser 2008).
Initial studies addressing the potential for miRNA regulation by BNDF examined the brain-specific miRNA miR-134, which is associated with learning and memory in rodents and negatively regulates spine size by inhibiting Limk1 mRNA in the synapse (Schratt et al. 2004; Gao et al. 2010). Limk1 undergoes increased translation in response to BDNF , and the authors found that BDNF prevents miR-134-mediated repression of Limk1 through an unknown mechanism (Schratt et al. 2004, 2006). BDNF was shown to decrease levels of miR-9, relieving suppression of MAP1b and leading to increased axon branching (Dajas-Bailador et al. 2012). In addition to negative regulation of miRNAs , BDNF was also reported to upregulate the transcription of several miRNAs. For example, miR-134 transcription was enhanced by BDNF, promoting inhibition of the RNA-binding protein Pumilio2 (Pum2). This caused an increase in dendritic arborization of young cultured neurons (Fiore et al. 2009; Vessey et al. 2010). Additionally, BDNF is thought to increase transcription of miR-132, a regulator of dendrite outgrowth and morphology, in a MAPK-dependent manner (Vo et al. 2005; Wayman et al. 2008; Kawashima et al. 2010). Interestingly, inhibition of miR-132 was shown to reduce BDNF-mediated upregulation of several synaptic glutamate receptor proteins such as NR2A, NR2B, and GluA1 (Kawashima et al. 2010).
In addition to these reports of BDNF effects on individual miRNAs , recent work from our laboratory has shown that BDNF can act upstream in processing steps to coordinately regulate the biogenesis of multiple miRNAs. Precursor miRNAs undergo several well-established processing steps to produce the functional mature miRNA. The canonical miRNA biogenesis pathway starts with transcription of a larger precursor miRNA (called a pri-miRNA) in the nucleus, where it is processed into a pre-miRNA stem-loop structure (of ~ 70 nucleotides) by the enzyme Drosha . This pre-miRNA hairpin is then exported into the cytoplasm, where it undergoes a second processing step by the RNaseIII enzyme Dicer and its binding partner Tar-RNA Binding Protein (TRBP) into a mature miRNA duplex. Regulation of either of these processing steps has the potential to dramatically affect the levels of functional mature miRNAs, and the translation of their corresponding mRNA targets. We demonstrated that BDNF achieves gene target specificity in protein synthesis by regulating the miRNA biogenesis pathway (Huang et al. 2012). BDNF stimulation of cultured hippocampal neurons elevates both Dicer and TRBP proteins in a rapid, transcription-independent manner, which enhances pre-miRNA processing and is associated with a concurrent increase in many mature miRNAs. This finding might initially appear somewhat counterintuitive, given that an increase in mature miRNAs could suggest a decrease rather than the modest increase in total translation produced by BDNF. However, we also observed that BDNF selectively decreases the processing of a specific class of miRNAs that are inhibited by the RNA-binding protein Lin28. The Let-7 family of miRNAs are a highly abundant group of these Lin28-targeted miRNAs, which repress a range of pro-growth mRNAs that are crucial for synaptic function, such as CamKIIα and GluA1 (Huang et al. 2012). The Let-7 class of miRNAs has received widespread attention due to their role in development and cancer. Because they inhibit translation of a range of important pro-growth proteins, the Let-7 miRNAs are virtually absent from stem cells, and are substantially upregulated during developmental progression (Moss et al. 1997; Loohuis et al. 2012). Additionally, Let-7 miRNAs function as tumor suppressor genes and are downregulated in roughly 15 % of cancers (Wang et al. 2012a). The Let-7 miRNAs are one of the most highly expressed classes of miRNAs in mature cell types, and high-throughput data suggests that Let-7 miRNAs make up at least 50 % of miRNAs present in mature neurons (Juhila et al. 2011; Shinohara et al. 2011). By reducing Let-7 levels and relieving Let-7 miRNA-mediated repression, BDNF is able to produce enhanced translation of a restricted subset of targeted mRNAs which presumably underlies the modest global increase in translation caused by BDNF.
Regulation of Let-7 miRNA biogenesis by Lin28 has been well documented (Moss et al. 1997; Heo et al. 2008, 2009; Nam et al. 2011). Lin28a and its mammalian paralog Lin28b are RNA-binding proteins and pro-growth pluripotency factors that are highly expressed in stem cells and a range of cancer cells and are downregulated during development, mirroring the increase in Let-7 miRNAs (Moss et al. 1997; Loohuis et al. 2012). This opposing expression pattern occurs through negative feedback between Lin28 and Let-7 miRNAs. Lin28 proteins recognize and bind a ‘GGAG’ motif in the terminal loop of Let-7 miRNA precursors, which causes degradation or inhibits processing of the precursor Let-7 miRNAs into mature miRNAs (Heo et al. 2008, 2009; Nam et al. 2011). Thus, Lin28 proteins are able to prevent Let-7 miRNA maturation prior to Dicer /TRBP processing. Lin28 mRNAs are, in turn, subject to repression by Let-7 miRNAs which can create feed-forward derepression when Lin28 proteins are elevated. Though Lin28 is generally considered to be absent from differentiated cell types such as neurons, we found that Lin28a protein was substantially elevated by BDNF in a rapid, transcription-independent manner, and that this accounted for the specific decrease in mature Let-7 miRNA expression following BDNF stimulation (Huang et al. 2012). Collectively, BDNF positively regulates general miRNA biogenesis by upregulating Dicer and TRBP proteins, and specifically negatively regulates Let-7 miRNA biogenesis in particular by increasing Lin28a protein. In this way, BDNF increases miRNA-mediated repression of many mRNAs while selectively relieving translational repression of mRNAs that are targets of Let-7 miRNAs. Increased miRNA biogenesis by Dicer/TRBP induction could account for the transcripts that have been shown to undergo decreased translation in response to BDNF (Rivera et al. 2002; Raab-Graham et al. 2006), and indeed knockdown of Dicer protein in cultured neurons could prevent BDNF-mediated decrease in such targets (Huang et al. 2012). In contrast, BDNF leads to increased translation of specific pro-growth mRNAs that harbor Let-7 miRNA binding sites by increasing Lin28a protein levels to inhibit Let-7 miRNA processing. Knockdown of Lin28a was validated to prevent a BDNF-mediated increase in multiple important Let-7 containing targets, such as CamKIIα, that are known to contribute to the synaptic and cognitive effects of BDNF (Huang et al. 2012). Further, synthetic insertion of a Let-7 miRNA binding site in an mRNA 3′UTR , was shown to be sufficient to create a BDNF-upregulated target gene.
5 Physiological Effects of Post-transcriptional Regulation by BDNF
5.1 Neuronal Morphology
Alterations in neuronal structure, such as dendritic outgrowth and spine maturation, are physiological components of enduring plasticity that have been shown to require BDNF -dependent changes in protein synthesis (Jaworski et al. 2005; Tanaka et al. 2008). In a translation-dependent manner, BDNF signals through TrkB receptors to enhance dendritic complexity and branching in both hippocampal and cortical neurons (Horch and Katz 2002; Jaworski et al. 2005; Cheung et al. 2007; Takemoto-Kimura et al. 2007; Tanaka et al. 2008; Je et al. 2009; Lazo et al. 2013). BDNF released from one neuron can enhance dendritic arborization of a closely neighboring neuron by binding to its TrkB receptors, and pyramidal neurons lacking TrkB receptors have significantly reduced pyramidal dendritic arbors (Horch and Katz 2002; Xu et al. 2000) This ability of BDNF to increase dendritic arborization is clearly linked to its regulation of translation. For example, Jaworski et al. (2005) demonstrated that enhanced dendritic complexity in response to BDNF expression was dependent on the mTOR signaling pathway, and could be blocked by inhibiting translation initiation. Studies from our laboratory have shown that the gene target specificity of BDNF-mediated translation is also crucial for its promotion of dendritic arborization. In particular, enhanced dendritic growth requires BDNF-induced reductions in Let-7 miRNA levels mediated by the RNA-binding protein Lin28a, presumably due to the many plasticity and growth-related mRNAs that are targeted by Let-7 miRNAs . In hippocampal neurons expressing a Lin28-resistant precursor of Let-7 miRNA, BDNF is no longer able to enhance dendritic arborization (Huang et al. 2012).
BDNF is also a well-characterized regulator of spine dynamics, and can induce changes in dendritic spine size and number. In cultured hippocampal neurons, BDNF has been shown to robustly increase spine density on the apical dendrite of pyramidal neurons in a MAPK/ERK-dependent manner (Tyler and Pozzo-Miller 2001, 2003; Alonso et al. 2004). Research has also suggested a differential effect of acute (one time) vs gradual (low-level repeated) BDNF stimulation on spine morphology. Specifically, acute BDNF stimulation of cultured hippocampal neurons produced transient TrkB activation and resulted in spine head enlargement, indicative of a more mature spine with a larger post-synaptic density. Alternatively, gradual BDNF stimulation led to long-term TrkB elevation and spine neck elongation, suggesting spine destabilization and remodeling. Both types of BDNF stimulation caused an increase in overall spine density (Ji et al. 2010).
Again, the effect of BDNF on spine plasticity is dependent on its regulation of protein-synthesis. In general, activity-induced upregulation of dedritic spines can be blocked by treating cultured neurons with the drug anisomycin, an inhibitor of protein synthesis (Fifková et al. 1982; Srivastava et al. 2012). More directly, long-term increases in hippocampal spine density mediated by BDNF have been shown to require new translation utilizing a system that allows inducible cell-autonomous inactivation of protein synthesis (Je et al. 2009). BDNF signaling through TrkB was also demonstrated to be necessary and sufficient for LTP -mediated spine head enlargement, and this effect was entirely blocked by anisomycin (Tanaka et al. 2008). Collectively, this research indicates that post-transcriptional regulation of gene expression by BDNF can induce structural changes in both synaptic and dendritic morphology that participate in plastic responses.
5.2 LTP and Memory
Synaptic plasticity is widely considered the cellular correlate of learning and memory, and involves the selective strengthening and weakening of synapses in response to incoming stimuli. The strengthening of specific synapses occurs through a phenomenon known as long-term potentiation (LTP ), and is the most widely studied and best understood form of synaptic plasticity . LTP involves sequential phases that include both early-LTP (E-LTP), which lasts 1–2 h and is transcription and translation independent, and late-LTP (L-LTP) which is of longer duration and is known to require novel transcription and translation (Krug et al. 1984; Stanton and Sarvey 1984; Costa-Mattioli et al. 2009). Interestingly, L-LTP can be blocked locally by restricted dendritic administration of protein synthesis inhibitors to prevent local translation (Bradshaw et al. 2003).
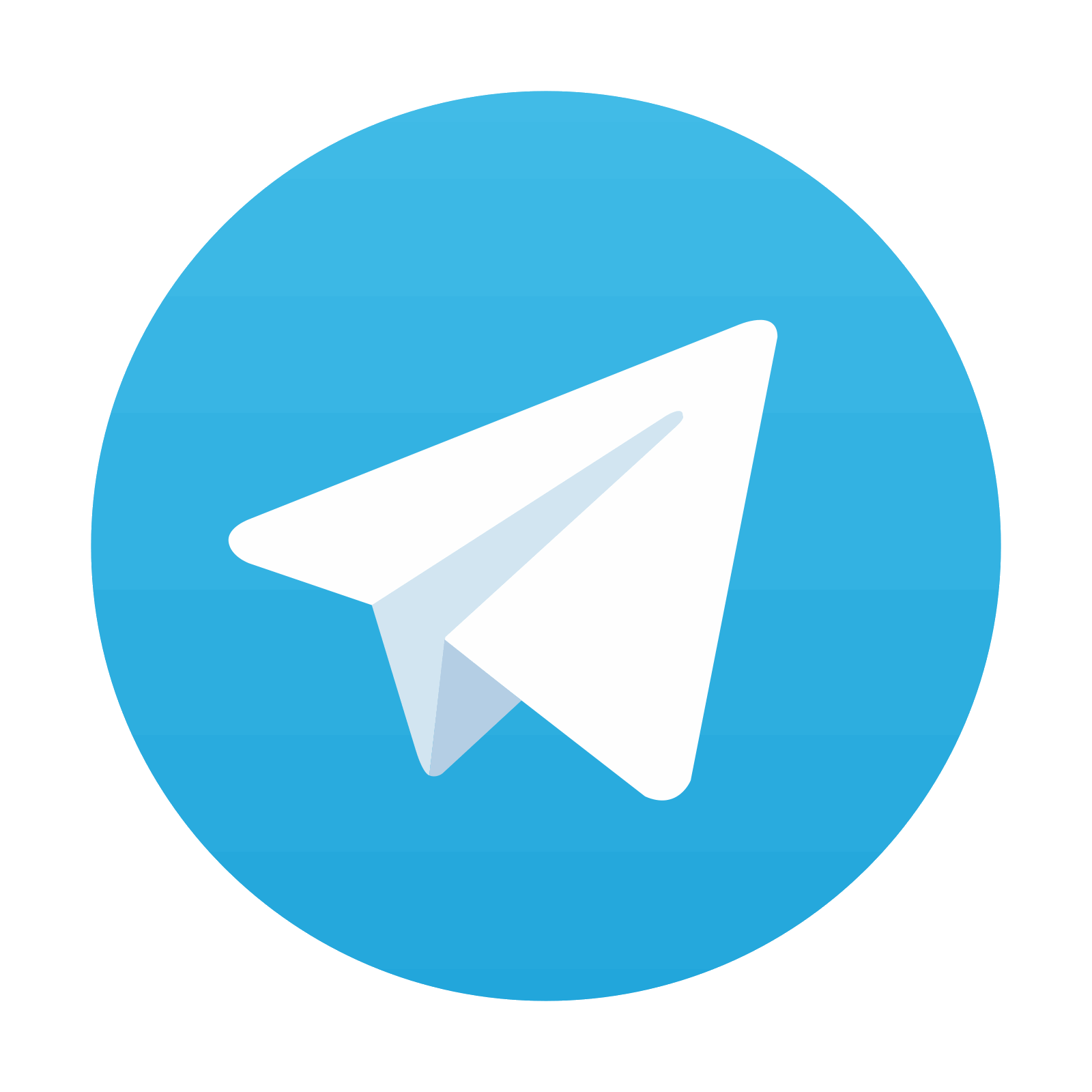
Stay updated, free articles. Join our Telegram channel
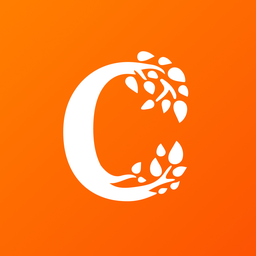
Full access? Get Clinical Tree
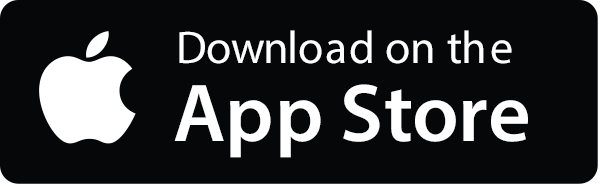
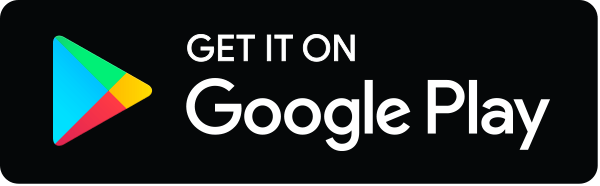