Pediatric Malignancies
Jeffrey C. Buchsbaum
Lee T. Myers
Introduction
In addition to the psychosocial issues, which require increased understanding and involvement, radiotherapy (RT) for pediatric malignancies differs from adult treatment in three fundamental physical and biological respects. First, many pediatric tumors are radioresponsive and require relatively low doses, especially as chemotherapy is also used. Second, growing tissues are likely to suffer more damage from RT than their adult counterparts. Third, there are immobilization issues with babies and young children that are not major factors in adult RT.
Treatment planning in pediatric RT is intimately involved with all three issues. In particular, target volumes are out of necessity well defined, with narrow margins, and therefore immobilization is the key. Developments in radiation therapy over the past 15 years have provided multiple tools and techniques that are especially useful with the pediatric population. Virtual simulation with multimodality image fusion (positron emission tomography [PET], magnetic resonance imaging [MRI], and magnetic resonance spectroscopy [MRS]), simplifies the simulation process and improves our ability to localize the target. The use of biological and molecular markers may significantly help us define target volumes. Dynamic multileaf collimators (MLCs) and automatic sequencing of table, gantry, and collimator motions have significantly enhanced our ability to quickly deliver complex treatment plans, which spare normal tissues and cover the necessary target. The use of three-dimensional conformal radiotherapy (3DCRT), intensity-modulated radiotherapy (IMRT), and more recently image-guided radiotherapy (IGRT) have allowed us to tailor dose and minimize margins necessary to treat the disease. Stereotactic radiosurgery (SRS), both linac-based and with the gamma knife, has allowed us to treat brain lesions with high conformality. Developments in stereotactic body radiotherapy (SBRT) are helping us to treat extracranial lesions with increased precision, which has obvious benefits for the pediatric population. In situations where integral dose may be an issue or where organ motion may be problematic, both low-dose-rate and high-dose-rate brachytherapy continue to offer practical alternatives. Finally, because protons can provide a more conformal physical dose distribution than photons, they may be especially useful for treating smaller targets in smaller patients and in optimizing integral dose (1,2). It is important to understand that the physics and dosimetry of protons in a clinical environment is less well tested over time than photons (3), and we may not fully understand the physics of protons in areas such as the sinuses and moving organs. We do not fully understand the biology of the proton at the Bragg peak. We also do not fully understand the risks of one form of proton beam as compared to another form of proton beam delivery system (4).
An open, critical pediatric radiation oncology question is “what is the long-term role of low-dose radiation therapy” in the form of integral dose as it is delivered with IMRT. By integral dose, we generally refer to the effects of the low-dose radiation bath delivered to a relatively large volume of tissue usually outside of the planned treatment volume. Formally, it is the sum of all doses to the entire body volumetrically. In the pediatric population, this needs to be studied prospectively. Some presentations at the American Society for Therapeutic Radiology and Oncology (ASTRO; Denver, 2005 meeting) evaluated the varied dose to organs in a pediatric phantom from 3D conformal and IMRT techniques. The results of the study highlighted both expected and unexpected issues and pointed to a need to design linear accelerators (LINACS) with less leakage from the head. Since the time of presentation, head leakage issues have been addressed by the manufacturers to varying degrees. It is imperative that centers and manufacturers monitor their designs and implementations to minimize dose to patients that do not come from the beam.
Integral dose needs to be considered in the treatment planning of pediatric patients as we move to increasingly higher usage of IMRT and IGRT (5). At present, it is critical that clinicians, dosimetrists, and physicists carefully communicate about this issue as we move forward with complex IMRT-based technologies that may in the end cause more secondary malignancies. In general, the increased doses and anatomic conformality allowed by IMRT and IGRT are likely to be more beneficial (6) than the cost of increased integral dose, but this is not yet backed by long-term data of 20 or more years duration (7). Image guidance further confuses the issues as very large volumes of tissue are often receiving low doses of radiation on a daily basis.
After a short discussion of immobilization, anesthesia, and image guidance, the various diseases (essentially different
from adult cancer) are discussed separately, as the issues concerned with each are diverse. Methods for treating and for treatment planning will be reviewed with special emphasis on new modalities and techniques. Tolerance of normal structures is not specifically covered here as it is beyond the scope and complexity of this chapter and it is well covered in Pediatric Radiation Oncology by Halperin, Constine, Tarbell, and Kun. The Children’s Oncology Group (COG) is currently looking at exploring this topic in a prospective fashion. Current protocols place goal doses for normal anatomy (often with the chemotherapy lowering normal tolerance dosing to some degree). This has been recognized by the leadership in radiation oncology in COG as an area of critical importance.
from adult cancer) are discussed separately, as the issues concerned with each are diverse. Methods for treating and for treatment planning will be reviewed with special emphasis on new modalities and techniques. Tolerance of normal structures is not specifically covered here as it is beyond the scope and complexity of this chapter and it is well covered in Pediatric Radiation Oncology by Halperin, Constine, Tarbell, and Kun. The Children’s Oncology Group (COG) is currently looking at exploring this topic in a prospective fashion. Current protocols place goal doses for normal anatomy (often with the chemotherapy lowering normal tolerance dosing to some degree). This has been recognized by the leadership in radiation oncology in COG as an area of critical importance.
If possible, children should be treated on protocols in the definitive setting. We are in an era of rapid technological change and we need to collect data on the new modalities of treatment. Some offer enormous promise but may hide enormous costs. In fact, our success in pediatric cancer has opened up a new horizon in pediatric radiation oncology—reirradiation. At present, there is little data in this realm, but the newest technologies will come to bear so as to minimize dose to tissue that has already seen some treatment in the past. Toxicity to wide margin re-treatment is high, so stereotactic body irradiation, proton therapy, and SRS will likely plan a role in this nascent field (8,9).
Immobilization of the Child
Children resist immobilization. As mentioned earlier, tumor targeting with accurate dose delivery is even more important with children than with adults. Fields are smaller, margins are tighter, anatomy is smaller, and critical structures are more sensitive. This dichotomy becomes a major problem in the radiation oncology of pediatric cancers. Often, the more children are restrained, the more they struggle to move. Consequently, one should carefully and practically immobilize only the region of the anatomy that requires immobilization. In the process of developing immobilization, child life personnel can help to make the child more comfortable with the devices and concepts of immobilization. In some instances, they can allow the avoidance of anesthesia (Fig. 32.1).
Bite blocks, thermoplastic masks, and body casts are good methods of ensuring accurate and consistent setups and beam delivery. Bite blocks and thermal masks are particularly good for head immobilization. Body casts can be made with plaster of Paris strips, polyurethane molds (Alpha Cradle), or vacuum bags (Vac-Lok). It may simplify future setups to allow the child ample opportunity to play with and get accustomed to the immobilization device. Although virtual simulation significantly reduces the time necessary for a child to endure the simulation process, it may be useful to have the child repeat the setup to check immobilization and to gain some additional
comfort with their device. A dry run may help make the “real” simulation proceed more smoothly. If targeting and immobilization become problematic, sedation should be considered. The simulation process can be significantly lengthened when cooperation of an unsedated child is required. Figure 32.2 shows the setup and immobilization of a child. Ample room must be available for the anesthesiology team to setup and do their work. Immobilization devices should always be constructed for nonsedated treatment because the need for anesthesia or sedation may decrease as the child adapts to the treatment process.
comfort with their device. A dry run may help make the “real” simulation proceed more smoothly. If targeting and immobilization become problematic, sedation should be considered. The simulation process can be significantly lengthened when cooperation of an unsedated child is required. Figure 32.2 shows the setup and immobilization of a child. Ample room must be available for the anesthesiology team to setup and do their work. Immobilization devices should always be constructed for nonsedated treatment because the need for anesthesia or sedation may decrease as the child adapts to the treatment process.
Anesthesia
Although modern, general anesthesia (GA) is essential for the treatment of babies and very young children, on a daily basis, it is time consuming and labor intensive,
requiring expertise and special equipment for patient monitoring (which can be a problem in some RT departments and adult hospitals) as well as for administration of the anesthetic.
requiring expertise and special equipment for patient monitoring (which can be a problem in some RT departments and adult hospitals) as well as for administration of the anesthetic.
Fortney, Halperin, Hertz, and Schulman (10) have given an excellent description and discussion of the subject, where they review the need for and application of anesthesia in 512 patients under the age of 16. Anesthesia is generally necessary for children under 3 years of age and rarely required for children over 5 years of age.
With the advent of IMRT and IGRT, treatment times are increasing. In the cross section of risk, it is generally felt that time under the effects of GA should be minimized if at all possible. In the design of pediatric plans, elapsed time needs to be evaluated carefully along with the same constraints as adults. Segmentation efficiency of planning systems is critical and research in this area will be critical to help minimize toxicity to the population of patients described in this chapter who are under GA. An IMRT plan generated and treated is shown in Figure 32.3. In its
initial form, this plan would have taken 30 to 40 minutes to deliver a dose. After several days of optimization, the same dosimetry was achieved in a 15-minute time slot. Newer techniques can deliver this treatment in minutes using arc therapy, but at the possible cost of integral dose. One often needs to be clever and use small partial arcs to gain a dosimetric advantage without a huge integral dose penalty. The radiation oncology team must balance integral dose against time on the table, conformality, and critical structure sparing. In some cases, a few well-chosen beams that do not pass through critical structures may provide a better result.
initial form, this plan would have taken 30 to 40 minutes to deliver a dose. After several days of optimization, the same dosimetry was achieved in a 15-minute time slot. Newer techniques can deliver this treatment in minutes using arc therapy, but at the possible cost of integral dose. One often needs to be clever and use small partial arcs to gain a dosimetric advantage without a huge integral dose penalty. The radiation oncology team must balance integral dose against time on the table, conformality, and critical structure sparing. In some cases, a few well-chosen beams that do not pass through critical structures may provide a better result.
Image Guidance
Although daily image guidance might significantly benefit the pediatric population, its use needs to be balanced against the long-term risks of increased radiation dose. In January 2008, the Alliance for Radiation Safety in Pediatric Imaging launched its Image Gently, Step Lightly campaign designed to reduce the radiation dose received by children during imaging procedures (11,12). In the past, children frequently underwent imaging procedures that were designed for adult patients, which generally delivered more radiation dose than was necessary to get the
required information. This campaign suggests that imaging procedures be appropriately child-sized, frequently cutting the dose in half to get the same level of information. A major advantage of image guidance is that it allows margins to be reduced and smaller target volumes to be treated to therapeutic doses. Imaging volumes, however, are generally somewhat larger than target volumes. Frequent imaging may introduce a significant dose to a larger volume. It is important for the radiation oncologist to consider this dose when imaging protocols are established. Optimal techniques delivering minimal dose to the smallest overall volume should be planned. Table 32.1 shows some expected (13) doses for various imaging techniques to be considered and includes data from the AAPM Task Group 75 report (14). Since imaging doses significantly depend upon x-ray energy, technique, and where the dose is delivered, it is difficult to compare or combine therapy and imaging doses. Table 32.1 is provided for general guidance and pediatric imaging protocols should be carefully established.
required information. This campaign suggests that imaging procedures be appropriately child-sized, frequently cutting the dose in half to get the same level of information. A major advantage of image guidance is that it allows margins to be reduced and smaller target volumes to be treated to therapeutic doses. Imaging volumes, however, are generally somewhat larger than target volumes. Frequent imaging may introduce a significant dose to a larger volume. It is important for the radiation oncologist to consider this dose when imaging protocols are established. Optimal techniques delivering minimal dose to the smallest overall volume should be planned. Table 32.1 shows some expected (13) doses for various imaging techniques to be considered and includes data from the AAPM Task Group 75 report (14). Since imaging doses significantly depend upon x-ray energy, technique, and where the dose is delivered, it is difficult to compare or combine therapy and imaging doses. Table 32.1 is provided for general guidance and pediatric imaging protocols should be carefully established.
Table 32.1 Imaging Doses from OBI (14) | |||||||||||||||||||||||||||||||||||
---|---|---|---|---|---|---|---|---|---|---|---|---|---|---|---|---|---|---|---|---|---|---|---|---|---|---|---|---|---|---|---|---|---|---|---|
|
Portal imaging practices within the COG were reviewed in a paper by Olch. They found that much could be done to reduce imaging dose to tissue outside the treatment volume. Their recommendations include: (1) use a single exposure when the area of interest is large enough to see reference anatomy, (2) minimize the additional area irradiated when a second exposure is necessary, (3) check record and verify parameters and light field shapes against treatment plan parameters and beam’s eye view, (4) image during treatment when possible, (5) use kV imaging rather than MV imaging when possible, and (6) use the minimum number of monitor units possible to get a readable image. Imaging is thought to add 0.3% to 12% more lifetime risk of a cancer. Something to keep in mind is the effective dose while flying in the continental USA on a commercial flight has been estimated to be 0.0003 cSv/h (15). The effective dose of a CT of the head (adult) is felt to be 0.15 cSv or about 50 times flying for 10 hours (16). An adult CT of the chest is felt to be about 0.54 cSv or about 180 times the dose from flying 10 hours (17). The effective doses of an abdominal CT scan for an adult, a young adult, and a child are 0.39, 0.44, and 0.61 cSv, respectively, in the literature (18). Newer CT scanners and more attention to this issue have made the current exposures lower. It is advised that a department measure their equipment and review the sequences used for all patients in the simulator and on the machine so as to achieve doses that are “as low as reasonably achievable (ALARA)” (19,20,21).
Leukemia
Clinical Overview
Acute lymphoblastic leukemia (ALL) is the most common cancer in children treated by the radiation oncologist, although the indications for radiation therapy have diminished in the past several years. Concerns about the long-term sequelae from radiation treatment and improvements in chemotherapeutic regimens have fueled the debate about the role of radiation therapy for ALL. Table 32.2 lists the indications.
Table 32.2 Indications for Irradiation in Acute Lymphoblastic Leukemia | ||||||
---|---|---|---|---|---|---|
|
Total body irradiation (TBI) is frequently given as part of the preparatory regimen for bone marrow transplantation.
Although it was originally given alone, TBI is much more effective when chemotherapy is also employed. Therefore, typical regimens consist of cyclophosphamide and TBI or cyclophosphamide and busulfan, as TBI is primarily employed for immunosuppression. TBI regimens are, therefore, part of allogeneic but not autologous transplantations. Although single-dose TBI can be employed, fractionated regimens are now generally used with or without lung shielding and electron boosts to the chest wall.
Although it was originally given alone, TBI is much more effective when chemotherapy is also employed. Therefore, typical regimens consist of cyclophosphamide and TBI or cyclophosphamide and busulfan, as TBI is primarily employed for immunosuppression. TBI regimens are, therefore, part of allogeneic but not autologous transplantations. Although single-dose TBI can be employed, fractionated regimens are now generally used with or without lung shielding and electron boosts to the chest wall.
Prophylactic cranial irradiation (PCI) used to be routinely prescribed as part of the consolidation of remission in all patients following the experience at St. Jude’s Hospital (22). Today, however, because of the potential late effects on pituitary (growth effects) and cerebral function (neurocognitive deficits), its use is quite variable among single institutions and cooperative groups. Improved long-term survival has caused additional concern about the development of secondary, radiation-induced malignancies (23). Although some use PCI in most patients, it has been virtually eliminated by others. Almost all centers have lowered the dose from 24 to 18 Gy at 1.5 Gy per fraction and lower doses are currently under investigation (24). However, all would agree that cranial irradiation—often craniospinal irradiation (CSI)—is indicated for overt central nervous system (CNS) leukemia (25,26). Currently, it is a standard of care to use cranial irradiation in patients that are CNS 3 (≥5 white blood cells [WBCs] per μL) and slow responders in high-risk populations. The current COG trial for high-risk ALL evaluates 12 Gy for slow early responders and 18 Gy for CNS 3 patients (COG trials AALL0232 and AALL0434). New data from St. Jude has been presented, which suggests that high-dose chemotherapy may be able to achieve equivalent cure, but without data regarding the long-term toxicity of chemotherapy or the salvagability of those patients, if they relapse.
Testicular irradiation is no longer given as prophylactic treatment, although it reduced the incidence of testicular relapse, because it had no influence on survival in one reported trial (27). Routine testicular biopsy on boys in remission at the end of therapy also used to be popular, with approximately a 10% to 15% yield (22). Approximately 60% to 70% of patients can be cured with intensive chemotherapy plus bilateral testicular irradiation to 24 Gy (27). All cases of overt testicular relapse must be treated with bilateral irradiation in addition to reinduction of chemotherapy, as relapses can occur in the contralateral testis. Although persistent disease sometimes occurs with 24 Gy in 12 fractions, this dose is generally recommended. Testicular radiation for those with testicular disease is part of the current high-risk COG trial and is given during consolidation at 24 Gy in 12 fractions (COG trials AALL0232 and AALL0434).
Palliative RT is very effective for the control of symptomatic masses causing pain, chloromas (most common in acute myeloblastic leukemia), and renal involvement. More often than not this is performed just before death, and the total doses of 2 to 6 Gy are usually highly effective.
Lesions such as histiocytosis X often respond to very low doses of radiation therapy. One typical dose scheme used is 9 Gy in six fractions. This low-dose approach limits long-term toxicity and allows follow-up dosing to more historical doses such as 30 Gy while still staying within most toxicity thresholds, ultimately without causing growth and hormonal dysfunction. 3DCRT is often sufficient to give very good dose distribution without requiring long treatment times.
Treatment Planning
Central Nervous System Irradiation
Whole-brain irradiation is most often accomplished with opposed lateral fields. The patient is treated supine unless the spine is also to be treated. The field size is set to encompass the whole brain, with the helmet technique to exclude the anterior part of the eyes. The optic nerve is a site for relapse and must be included, as must the cribriform plate. This results in tight ports in the superior and posterior orbit. Setting the center of the field to the posterior orbit and blocking to protect the anterior portion of the orbit also eliminates beam divergence to the opposite eye. The radiation beam energy usually employed is 6-MV x-rays, although 4-MV or cobalt teletherapy can be used as effectively for most children. Lens dose can also be reduced by rotating the beam posteriorly ∼5 degrees (28). Virtual simulation is crucial once the anatomy is contoured as patients are often asymmetrical.
If it is necessary to match the brain field with a spinal field, the patient is generally treated in the prone position, and the couch can be kicked for each lateral brain field to produce a nondivergent edge at the inferior border. This edge can be matched to the top of the spinal field with a small gap (0.5 cm or less). The angle of the couch kick is arctan (L/2 × SAD), where L is the length of the brain field and SAD is the source-to-axis distance of the treatment machine.
The spinal field in most children can be treated with a single (posteroanterior [PA]) field. Therefore, the match to the brain field becomes the prime issue. Rotating the couch to a 90 degree position and rotating the gantry to direct the upper edge of the spinal field straight up can achieve a long field with a good match. The gantry angle is given by the arctan mentioned earlier, where L is the length of the spinal field. Alternatively, if the spinal field is small, a half-beam block can be used.
If the spinal field and the brain field must be matched, it is wise to migrate the border of the match at least twice during the course of treatment. The benefit to migrating the match line is shown in Figure 32.4. Including three match line changes improves the dose uniformity by ∼15%. An excellent discussion of craniospinal field matching techniques is given in Chapter 13 of The Physics of
Radiation Therapy (29). A review of the techniques used by Pediatric Oncology Group (POG) participants showed a surprising diversity in methods. Match line feathering was used by most institutions. Both prone and supine methods have been published, and one method that developed at the Cleveland Clinic avoids junction shifts by the use of wedges to “smear” the dose at the junction (30). The latter places an isocenter at the junction. Care must be employed to make the dose to junctioned areas equal, as unexpected dosimetry can result if the dose to adjacent areas is different (31).
Radiation Therapy (29). A review of the techniques used by Pediatric Oncology Group (POG) participants showed a surprising diversity in methods. Match line feathering was used by most institutions. Both prone and supine methods have been published, and one method that developed at the Cleveland Clinic avoids junction shifts by the use of wedges to “smear” the dose at the junction (30). The latter places an isocenter at the junction. Care must be employed to make the dose to junctioned areas equal, as unexpected dosimetry can result if the dose to adjacent areas is different (31).
Modern IMRT techniques allow the match to be optimized using either forward or inverse planning. The spinal field is designed to overlap with the brain field and the dose uniformity to the spine is set as an objective. The inverse planning engine will then derive optimal segmentation on the spinal field to optimize the field matching. This method allows the same fields to be treated each day with appropriate field segmentation, but may not necessarily provide the setup error tolerance of more conventional techniques.
Spinal cord irradiation has also been done with a single posterior electron-beam portal (32). In this technique the electron energy is chosen so that the 90% isodose line falls ∼7 mm anterior to the anterior cord. Bolus is designed to treat uniformly to this depth. The concern with electron irradiation of the spine is the effects of dose inhomogeneity associated with the presence of higher density bone (33). Although the electron technique may work well with very young patients, it may underdose patients when the depth of the cord exceeds 6 cm. Combinations of photons and electrons may sufficiently treat the cord and spare anterior structures. Careful planning that allows combinations of photons and electrons can determine optimal beam weighting to accomplish this treatment (34). The use of protons has enormous promise as it may allow the blunting of most of the gastrointestinal (GI) toxicity associated with CSI and decrease the integral dose of the child in the spinal regions significantly (35,36). Currently, most centers outside Switzerland have used passive scattering to shape fields and that has caused some concern due to neutron generation. The long-term effects of this are unknown, but there has not been a report of unusually high second malignancy in those treated with passively scattered protons. Scanning proton therapy eliminates neutron generation from materials placed in the primary beam.
Most treatment planning systems provide a sagittal view of dose along the craniospinal axis. When dose variations along the spinal cord exceed 10%, compensators or beam segmentation can be designed and used to provide a more uniform dose to the craniospinal axis. Some institutions prefer compensators and others use beam segmentation. This is an issue of spatial versus temporal intensity modulation. Either method works.
Testicular Irradiation
Irradiation of the testes in pediatrics is most often done with a single anteroposterior (AP) field set to the smallest field of the accelerator. If a photon beam is used, it can be angled in the superior–inferior direction treating the testes tangentially. If an electron beam is used, it is setup en face
to treat the testes directly. The penis is taped to the abdomen and out of the treatment field. The patient is placed in a frog-leg position. The scrotal sack may be taped to minimize the size of the electron cone needed. In all cases, a taping technique is used to avoid applying adhesive to the scrotal sack and penis shaft. The testes should be centered in the field, and a bolus may be applied to bring the center of the testes to the depth of maximum dose. The field may be reduced as the testicular mass decreases in size during therapy and electron energy should be chosen to minimize dose beyond the target if possible. Testicular boost in high-risk ALL patients can be added as part of TBI in the form of two 2-Gy fractions and has been employed at St. Jude on PM fractions of days 3 and 4.
to treat the testes directly. The penis is taped to the abdomen and out of the treatment field. The patient is placed in a frog-leg position. The scrotal sack may be taped to minimize the size of the electron cone needed. In all cases, a taping technique is used to avoid applying adhesive to the scrotal sack and penis shaft. The testes should be centered in the field, and a bolus may be applied to bring the center of the testes to the depth of maximum dose. The field may be reduced as the testicular mass decreases in size during therapy and electron energy should be chosen to minimize dose beyond the target if possible. Testicular boost in high-risk ALL patients can be added as part of TBI in the form of two 2-Gy fractions and has been employed at St. Jude on PM fractions of days 3 and 4.
Local Recurrence or Site-Related Disease
Treatment of local, isolated disease, whether nodal or otherwise, is site specific. In most children, individual sites can be treated with a simple AP/PA technique, with appropriate blocks for critical structures. If the spinal column is in the fields, the entire width of the vertebral body should be included. Similar clinical issues need to be addressed case by case. Although 3DCRT or IMRT may play a role in dealing with site-related disease, this role still needs to be determined and may be very site specific. Often, the simpler AP/PA approach may be sufficient.
Total Body Irradiation
TBI in children is very similar to that in adults. Doses of 12 Gy over 3 to 4 days in six to eight fractions is sufficient for immunosuppression. Dose is prescribed to midplane, typically at the level of the umbilicus. Compensators or bolus materials are used to maintain a dose uniformity of better than ±10% (Fig. 32.5).
A variety of treatment techniques have been used successfully (37,38). The advantage of the bilateral technique is that the arms shield the heart and lungs. The disadvantage is the general need for bolus or specially designed compensators. The AP/PA technique is inherently simpler but often requires whole or partial blocks to shield the heart and lungs. In general, higher-energy beams also improve dose uniformity but often require a spoiler or bolus to increase skin dose. A popular regimen is 12 Gy in six to eight fractions over 3 to 4 days. Institutions around the country have individualized the delivery of TBI. Lung blocking is typically employed lowering the midplane lung dose to 8 to 10 Gy. Computed tomography (CT) data can be useful for accurate lung dosimetry and block design (thickness). The cross section of lung blocking is a clinical decision made using treatment ports in many centers. New delivery device technology, such as TomoTherapy, may prove useful for more complex and accurate lung blocking and dosimetry (39). Currently, the use of MLC dose compensation and the dosimetry of the use of arms to block the lungs in lateral techniques has been studied using 3D planning systems and is the focus of clinical research (40). It is advantageous to keep the dose rate under 10 cGy per minute to help decrease emesis. Large treatment rooms are generally necessary for larger patients and are an advantage even with smaller patients. In addition to dose rate, immobilization is important because treatment can last up to 40 minutes or more. Institutions use various methods of immobilization; discussion of the merits of each type of device or positioning tool goes beyond the scope of this chapter.
Small children and infants require smaller fields and have relatively little variation in thickness throughout their anatomy. In addition, they often provide much less scatter than adults. Therefore, they can often be treated at
an extended distance with a lower-energy beam. Consequently, many centers use a different TBI technique for children. Infants can be treated on the floor with the gantry at zero degrees, underneath a spoiler that may also support lung blocks. The infant can be flipped from the supine to the prone position to allow an AP/PA treatment.
an extended distance with a lower-energy beam. Consequently, many centers use a different TBI technique for children. Infants can be treated on the floor with the gantry at zero degrees, underneath a spoiler that may also support lung blocks. The infant can be flipped from the supine to the prone position to allow an AP/PA treatment.
Regardless of the technique, TBI should be set up as a special procedure with attention to the limitations of the room and equipment, as well as the nature of the patient. Most treatment planning systems do not permit treatment planning at the extended distances necessary for whole-body irradiation.
Individual patient dosimetry is important for monitoring the overall dose uniformity during treatment. Typically thermoluminescent dosimeters (TLDs) or diodes are used for the first or the first few treatments. Entrance and exit doses are measured at several points along the patient’s anatomy. Adaptive RT was developed from the necessary improvements in TBI irradiation. Total dose or dose compensation can be adjusted as necessary. Subsequent measurements can be used to check setup reproducibility. It is not always possible to get a very homogeneous plan based on a child’s anatomy. In these situations, it is often best to use lung dose and then normal tissue toxicity to guide treatment design. Compromises are almost always necessary in TBI.
Lymphomas in Children
Hodgkin’s Disease
Clinical Overview
The disease process is essentially the same as in adults. For children, the concern is to provide the appropriate treatment for the minimum cost in late effects. Hodgkin’s disease is predictably radioresponsive, chemoresponsive, and frequently curable by either modality, alone or in combination, allowing for choice in management. Since current long-term survival rates may approach 97%, concerns about long-term sequelae with radiation treatment, including radiation-induced second malignancies, are very real.
In the adult, total nodal irradiation up to 35 Gy may be used, but the potential sequelae are such that this is almost always inappropriate in children. Primary chemotherapy, usually followed by involved-field RT to a reduced dose (15–25 Gy), is often preferred. The disease is predictable in its propensity to relapse in unirradiated, initially involved sites, and therefore involved-field RT is used. Assuming complete remission has been obtained by chemotherapy, the reduced dose is appropriate on the reduced tumor burden. Occasionally, a child is treated with involved-field RT only (e.g., pathologically staged 1A midneck), but in that situation full-dose (25–40 Gy) RT must be employed. Adolescents who have passed through puberty may be treated as adults with extended fields to full doses. Overall results are comparable with those of adult therapy, but pediatric dosing is lower and is likely to lead to lower dose standards for adult Hodgkin’s disease.
Treatment Planning
Involved-field RT in children requires precise staging and localization of involved nodes. Most treatments are done with an AP/PA technique to a reduced dose. The most common site is the neck and supraclavicular nodes.
For children past the age of puberty, extended-field RT can be used as in an adult. For supradiaphragmatic presentations, this usually includes a tailored mantle field followed by a para-aortic field treated to the level of L4. For subdiaphragmatic presentation, this usually includes para-aortic fields and inguinoiliac fields as an inverted Y.
The role of treatment planning in Hodgkin’s disease has been reviewed (41). Particularly when wide-field irradiation is employed, it is important to calculate and carry doses to a number of points throughout the treatment field. The AP/PA technique, with appropriate custom blocking of critical structures, such as the lung, is preferred. Inhomogeneities should be included, especially in mantle fields. Dose compensators or beam segmentation should be used if the dose variation exceeds ±7.5% or per protocol guideline. The general practice in adults is not to have special immobilization devices, but these are frequently necessary with children. In the case of subdiaphragmatic presentations and the use of the inverted-Y technique, gonadal shields are not generally used, especially if the gonads are not close to the field. Nonetheless, for adolescents, the use of gonadal shielding is more likely to be advised, as the issues of parenting are critical. The use of TLDs or diodes as an in vivo dosimeter is advised in the treatment of children. Currently, early stage disease fields in favorable patients are shrinking. The general move is to use risk-adapted algorithms to determine treatment portal design. The extreme of this can be seen in the current series of studies in Europe (42,43,44,45). Hodgkin’s disease is under intense study in terms of late effects on children, so dose and technique are likely to change in time so as to continue the high cure rates while hopefully decreasing toxicity (46).
The newest protocols coming out of COG for Hodgkin’s now allow 3D conformal and IMRT treatment using photons and electrons. Radiation is delivered in a “risk adapted” fashion. The dose is still 21 Gy at 1.5 Gy per fraction. Current COG protocols require central review. All treatment plans are submitted electronically and must be reviewed prior to treatment.
Non-Hodgkin’s Lymphoma
Clinical Overview
The spectrum of non-Hodgkin’s lymphomas (NHL) in children is different from that of adult NHL. In childhood,
nodular histology is very rare and extranodal presentations are common. Management by RT alone has only historical interest, as the cure rate even in local disease is ∼50%. With modern therapy, most patients are curable.
nodular histology is very rare and extranodal presentations are common. Management by RT alone has only historical interest, as the cure rate even in local disease is ∼50%. With modern therapy, most patients are curable.
Table 32.3 Role of Radiotherapy in Childhood Non-Hodgkin’s Lymphoma | ||||||||||
---|---|---|---|---|---|---|---|---|---|---|
|
Simplistically, NHL is divided into lymphoblastic and nonlymphoblastic disease, with both forms being managed primarily with chemotherapy. For childhood lymphoblastic disease, leukemia-style regimens based on lymphosarcoma2 leukemia2 Leukemia2 (LSA2L2) popularized by Norma Wollner at Memorial Sloan-Kettering (47) have been used successfully by (among others) the Children’s Cancer Group (CCG) (48). This same study showed that for nonlymphoblastic disease an intermittent regimen of cyclophospha mide, vincristine, methotrexate, and prednisone (COMP) was superior to LSA2L2. Initially, all patients received local RT along with chemotherapy, but more recent results from the POG show that in 129 patients there was no difference in the pattern of relapse or survival (93%) for patients with local NHL treated with chemotherapy with or without (27 Gy) local RT (49).
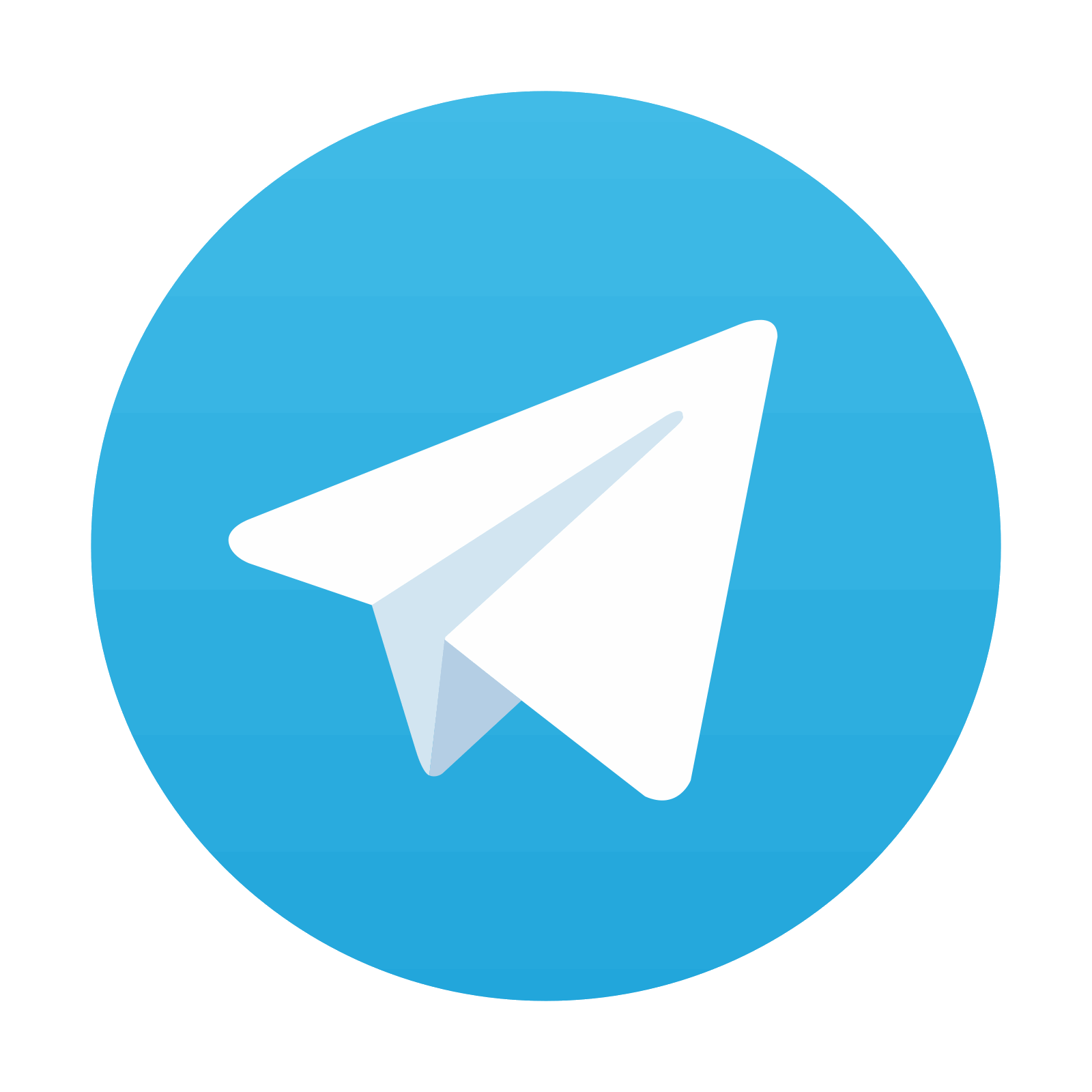
Stay updated, free articles. Join our Telegram channel
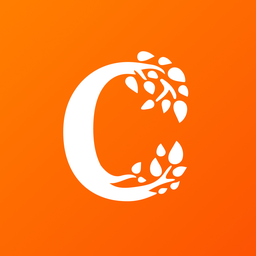
Full access? Get Clinical Tree
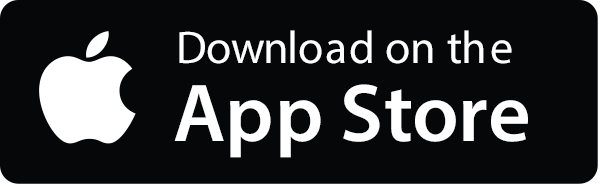
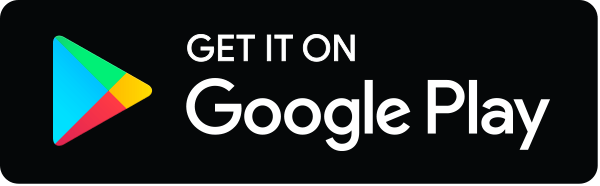
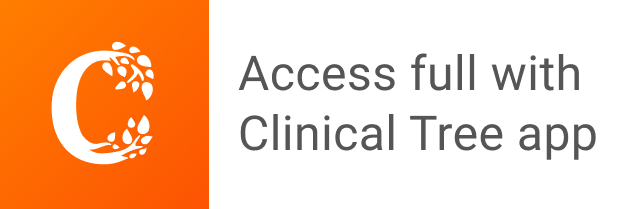