Chapter Outline
Paroxysmal Nocturnal Hemoglobinuria and Complement
Membrane Inhibitor of Reactive Lysis
Inherited Mutations of CD55 and CD59
Paroxysmal Nocturnal Hemoglobinuria and Deficiency of Glycosyl Phosphatidylinositol–Anchored Protein
A Two-Step Model of Paroxysmal Nocturnal Hemoglobinuria Pathogenesis
Medical textbooks usually include paroxysmal nocturnal hemoglobinuria (PNH) among the hemolytic anemias (as is the case herein). Doing so is not wrong because hemolysis is a defining feature of the disease. However, placing too much emphasis on the hemolytic component of the disease risks obscuring the essence of PNH. Two features distinguish PNH from all other hemolytic anemias. First, and most importantly, the abnormality that underlies the pathobiology of PNH is not confined to the erythrocyte. Rather, PNH is a disease of the hematopoietic stem cell. Secondly, PNH differs from all other intrinsic red cell abnormalities in that the defective process is acquired rather than inherited.
PNH arises as a consequence of somatic mutation of a gene (phosphatidylinositol glycan class A [ PIGA ]) whose protein product is a glycosyl transferase that is an essential component of the biosynthetic pathway that generates glycosyl phosphatidylinositol (GPI) ( Fig. 14-1 ). This moiety serves as the anchoring mechanism for a functionally diverse group of membrane-bound proteins, more than 20 of which are expressed on hematopoietic lineage cells in humans ( Box 14-1 ). The PIG-A mutations that give rise to PNH cause loss of enzyme function, the end result of which is the complete or near-complete absence of expression of all proteins that are GPI-anchored ( Fig. 14-2 ). Among the cellular membrane constituents that are GPI-anchored are the complement inhibitory proteins, CD55 (decay accelerating factor) and CD59 (membrane inhibitor of reactive lysis). It is deficiency of these two proteins that underlies the complement-mediated intravascular hemolysis characteristic of PNH ( Fig. 14-3 ). Despite the loss of all GPI-anchored proteins (GPI-APs) from all hematopoietic lineage cells (erythrocytes, granulocytes, monocytes, lymphocytes, and megakaryocyte/platelets) derived from the PIGA mutant stem cell, there is no compelling evidence that deficiency of GPI-APs other than CD55 and CD59 contribute significantly to the pathophysiology of PNH. In addition to complement-mediated intravascular hemolysis, the other major clinical manifestations of PNH are bone marrow failure and thrombophilia. While the relationship between somatic mutation of PIGA and the hemolysis of PNH is understood in detail, the relationship between somatic mutation of PIGA (and the consequent deficiency of GPI-APs) and the bone marrow failure and thrombophilia of PNH remain largely speculative. Especially in the case of bone marrow failure, the PIGA mutation/deficiency of GPI-APs may not be causal. In fact, the prevailing hypothesis posits that the process that causes the bone marrow failure (proposed as immune attack on the hematopoietic stem cell) selects for the PIGA mutant stem cell because absence of one or more GPI-APs bestow a survival advantage by allowing the mutant stem cell either to evade or to better weather immune attack. Whether the thrombophilia of PNH is due to absence of a GPI-AP that is directly involved in regulation of hemostasis/thrombosis (e.g., the receptor for urokinase plasminogen activator) or to indirect consequences of GPI-AP deficiency (e.g., generation of procoagulant microparticles as a result of complement-mediated lysis) remains unresolved.
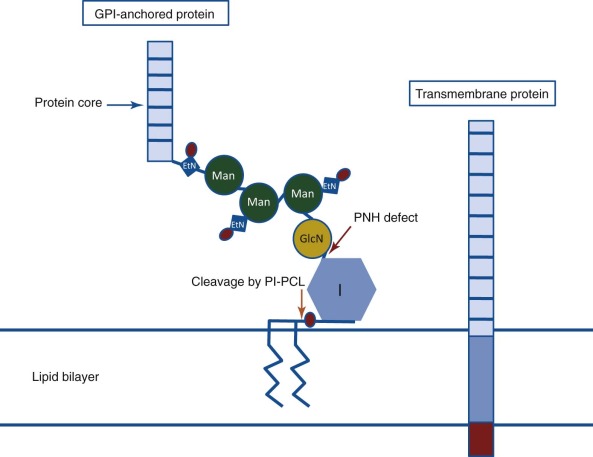
Complement Regulatory Proteins †
† Deficiency of complement regulatory proteins underlies the hemolytic anemia of PNH.
- •
CD55 (decay accelerating factor)
- •
CD59 (membrane inhibitor of reactive lysis)
Proteins with Immunologic Significance
- •
CD58 (lymphocyte function antigen-3)
- •
CD16b (Fc receptor gamma IIIb)
- •
CD14 (endotoxin binding protein)
Receptors
- •
CD87 (urokinase plasminogen activator receptor)
- •
Folate receptor
- •
Cellular prion protein (on resting platelets)
Enzymes
- •
Leukocyte alkaline phosphatase
- •
Acetylcholinesterase
- •
5′-ectonucleotidase
Miscellaneous Proteins
- •
CD24
- •
CD48
- •
CD52 (Campath-1)
- •
CD66c
- •
CD66b (formerly CD67)
- •
CD90 (Thy-1)
- •
CD108 (JMH-bearing protein)
- •
p50-80, GP109, GP157, GP175, GP500
PNH, Paroxysmal nocturnal hemoglobinuria.
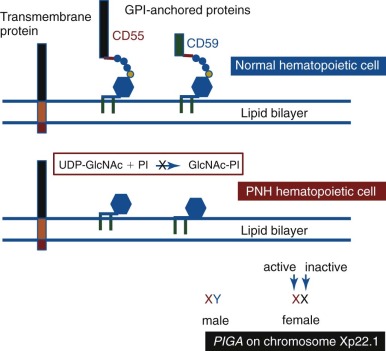
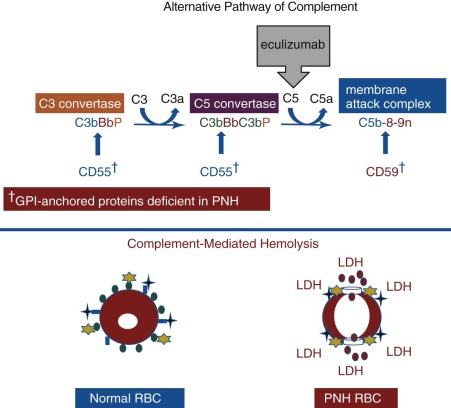
PNH is a clonal disease (mutant PIGA serves as the clonal marker), but it is not a malignant disease in the sense that acute myeloid leukemia (AML) or chronic myelogenous leukemia (CML) are malignant clonal diseases of the hematopoietic stem cell. Unlike AML or CML, in which the mutant clone expands unrelentingly unless antileukemic therapy is initiated, the extent to which the PNH clone expands varies greatly among patients. For example, some patients have small PNH clones that can be detected only by using high-sensitivity flow cytometry to identify GPI-AP peripheral blood cells. Other patients may have large clones in which essentially all hematopoiesis is derived from the mutant stem cell; but even when large, the PNH clone does not have malignant properties in that it does not invade sites outside of the bone marrow, nor does it behave autonomously (i.e., its functional properties appear to be governed by the same regulatory factors as those that control the functional properties normal stem cells), and transformation into acute leukemia is rarely observed. In fact, an unsolved mystery of PNH is what determines the extent to which the PIGA -mutant clone expands. This question is framed by in vitro and in vivo studies demonstrating that mutant PIGA alone is insufficient to account for clonal expansion.
More than 25 proteins are involved in the synthesis of the GPI-anchor moiety. Hypothetically, somatic mutation of any of the genes that encode these proteins would produce the PNH phenotype (i.e., deficiency of GPI-APs). Why then is PNH essentially always due to mutation of PIGA ? The putative answer is based on mathematical probability. Among the genes involved in GPI-AP synthesis, PIGA alone is located on the X chromosome. Therefore only one mutational event is required to produce the PNH phenotype because males have one X chromosome, and in females, only one of the two X chromosomes is active in somatic tissues (see Fig. 14-2 ). Because all other genes involved in GPI-AP synthesis are autosomal, two mutational events would be required to eliminate synthesis of the GPI-anchor and produce the PNH phenotype. Thus, based on mutational frequency (~1.1 × 10 −8 per nucleotide position per haploid genome), the probability that deficiency of GPI-APs in PNH would be due to somatically mutated PIGA is many orders of magnitude greater than the probability that the deficiency would be due to somatic mutation of both of the alleles of any of the more than 25 autosomal genes involved in GPI-anchor synthesis. Conceivably, germline mutation of one of the alleles of an autosomal gene involved in GPI-AP synthesis could predispose an individual to develop an autosomal form of PNH, and a recent report supports this mechanism as a rare cause of the disease. In essence, however, this situation is analogous to somatic mutation of the X-chromosome gene PIGA because the patient was functionally hemizygous for the autosomal gene ( PIGT ) because of the germline mutation of one of the two alleles. This observation adds additional support to the hypothesis that mutant PIGA underlies PNH in essentially all cases of PNH because of its unique property among the genes involved in GPI anchor synthesis of structural or functional hemizygosity in somatic tissues.
Understanding the molecular basis of the hemolysis of PNH led to development of targeted therapy in the form of eculizumab (Soliris, Alexion Pharmaceutical), and this treatment has had a major impact on the natural history the disease. Nonetheless, management of patients with PNH remains challenging because of the heterogeneous nature of the disease and its close association with bone marrow failure.
This chapter is intended to illuminate for the reader the pathobiology of PNH and to provide guidance on the diagnosis and management of this fascinating disease.
History
Comprehensive, scholarly reviews of the history of PNH have been published. The renown Victorian physician, William Gull, may have provided the first published clinical description in 1866, although his brief report failed to distinguish unequivocally PNH from paroxysmal cold hemoglobinuria, which was relatively common at that time because of its relationship to syphilis. In 1882, Paul Strübing of Greifswald, Germany clearly recognized PNH as a distinct entity and undertook prescient experiments designed to test his hypothesis that the nocturnal hemoglobinuria was a consequence of acidification of plasma that occurred when carbon dioxide and lactic acid accumulated because of slowing of respiration during sleep. In 1911, the prolific Dutch physician A.A. Hijmans van den Berg demonstrated that the hemolysis of PNH is caused by a defect in the red cell rather than by the presence of an abnormal plasma factor (as is the case with paroxysmal cold hemoglobinuria that is mediated by the Donath-Landsteiner antibody). Thomas Hale Ham, then in Boston, is credited with discovering, in the late 1930s, that complement mediates the hemolysis of PNH erythrocytes, although it was not until the alternative pathway of complement (APC) was identified and characterized in the mid-1950s by Louis Pillemer of Case Western Reserve University in Cleveland, Ohio, that the basis of Ham’s original observations became apparent. Notably Ham was on the faculty of Case Western when the alternative pathway was discovered, and Pillemer used PNH erythrocytes in some of his experiments that demonstrated the antibody-independent nature of the alternative pathway and its dependence on magnesium. While still in Boston, Ham developed the acidified serum lysis test (Ham’s test) that, along with the sucrose lysis test (sugar water test) of Robert Hartmann and David Jenkins of Vanderbilt University in Nashville, Tennessee, were used as the standard diagnostic tests for PNH until being supplanted in the mid-1990s by flow cytometry. Both Hartmann and William Crosby brought attention to the important role that thrombosis (particularly the Budd-Chiari syndrome) plays in the natural history of PNH, and the eminent English physician, John Dacie, and his pupil and colleague S.M. Lewis first systematically characterized the relationship between PNH and marrow failure. Other landmark events in the history of PNH include characterization of the remarkable phenotypic mosaicism, based on complement sensitivity in the late 1960s and early 1970s; demonstration that PNH is a disease of the hematopoietic stem cell in the late 1960s; proof of the clonal nature of the disease in the early 1970s; characterization of the erythrocyte complement defects in the 1970s and 1980s; identification of the complement regulatory proteins that are deficient in PNH in the 1980s; recognition that the membrane proteins that are deficient in PNH are GPI-anchored in the 1980s; identification of PIGA as the gene that is mutant in PNH in the 1990s; characterization of the genetic basis of the phenotypic mosaicism in the 1990s; and clinical testing and government approval of the complement C5–inhibitor eculizumab for treatment of the hemolysis of PNH in the first decade of the twenty-first century.
Epidemiology
Interpretation of prevalence studies of PNH must take into account the heterogeneous nature of the disease. The blood of patients with PNH is a mosaic of normal and abnormal cells, and the degree of mosaicism varies widely among patients. Those with small PNH clones have few or no symptoms related to hemolysis, and those with clones of less than 1%, detected by high-sensitivity flow cytometry, lack even biochemical evidence of hemolysis. Thus, an argument can be made that asymptomatic patients with small clones do not have clinically significant PNH and should be excluded from prevalence estimates. Others, however, may argue that any patient with flow cytometric evidence of a population of GPI-AP–deficient (GPI-AP−) cells, regardless of clone size, has PNH and should be included in prevalence statistics. Studies of prevalence that address the issue of disease heterogeneity are needed, but by any definition PNH is a rare disease. The prevalence of clinically significant PNH is likely in the order of less than 1 case per 200,000 population, easily fulfilling criteria (less than 1 case per 50,000 population) for classification as an ultraorphan disease.
There is a close association between PNH and aplastic anemia (AA). Environmental factors, drugs, and toxins that cause AA concordantly increase the risk of developing PNH. Although PNH has been reported in all age groups, the peak incidence is in the third and fourth decades of life, similar to that observed for AA. PNH is an acquired disorder, and there is no known inherited risk for developing the disease. A number of cases have been reported in which only one of a pair of identical twins was affected. Inherited mutations in autosomal genes involved in GPI anchor biosynthesis have been identified, but patients with such germline mutations (homozygotes or compound heterozygotes) are characterized by developmental and neurologic abnormalities, including mental retardation and seizures, and by hyperphosphatasia. A patient with mutant PIGA died in early infancy because of multiple developmental defects without evidence of hemolysis. Based on this observation and animal studies, PIGA mutations whose consequences are sufficiently severe to render the red cells susceptible to complement-mediated hemolysis are embryonic lethal.
Molecular Pathogenesis
Careful in vivo studies of red cell survival and in vitro characterization of the complement sensitivity of peripheral blood erythrocytes hinted at the molecular basis of PNH. Red cell survival studies suggested that two populations of erythrocytes coexisted in the peripheral blood of patients with PNH. One population had an abnormally short half-life of about 6 days, whereas the other population had a half-life similar to that of normal erythrocytes (about 60 days). Those observations dovetailed with the results of rigorous experiments that quantified the sensitivity of peripheral blood erythrocytes to complement-mediated lysis, showing that the peripheral blood of PNH patients is a mosaic composed both of erythrocytes that are normally sensitive to complement-mediated lysis and of red cells that are abnormally sensitive to complement-mediated lysis. Together these observations lead to the hypothesis that the abnormal cells were the progeny of a mutant hematopoietic stem cell clone that produced the abnormal erythrocytes. According to this hypothesis, the mutant stem cell coexisted within the bone marrow with normal stem cells from which the erythrocytes of normal complement-sensitivity were derived. Further, that PNH is an acquired rather than an inherited disease implied that the abnormal clone arises as a consequence of somatic mutation rather than germline mutation. In 1970, Oni and colleagues analyzed glucose-6-phosphate dehydrogenase (G6PD) isoforms in both the complement-sensitive and the complement-insensitive erythrocytes of a female PNH patient who was heterozygous for the two G6PD isoforms. The complement-insensitive cells expressed both G6PD isoforms, indicating a polyclonal origin for this cohort. In contrast, the complement-sensitive cells expressed only one G6PD isoform, a finding consistent with monoclonality. These studies provided the first experimental evidence in support of the clonal hypothesis of PNH. In 1969, Aster and Enright reported that a portion of both the platelet and granulocyte populations from patients with PNH was abnormally sensitive to complement-mediated cytolysis. This publication represented another watershed event in the understanding of the origins of PNH because it indicated that the mutation arose in a primitive hematopoietic stem cell that has the capacity to differentiate along myeloid lines. Subsequent studies showed that monocytes are also affected, and, in most patients, affected lymphocytes can be demonstrated. Thus, characterization of PNH contributed substantial early experimental evidence in support of the concept of the pluripotent stem cell.
A defining feature of PNH is phenotypic mosaicism based on sensitivity of the erythrocytes to complement-mediated lysis. This remarkable characteristic was first clearly elucidated by Rosse and Dacie in 1966, and Rosse further refined the analysis in 1973. Using an in vitro test that quantitates the sensitivity of erythrocytes to complement-mediated lysis, three phenotypes of PNH erythrocytes were identified. One of the phenotypes (designated PNH I) was characterized by normal or near-normal sensitivity to complement, whereas another phenotype (designated PNH III) was 15 to 25 times more susceptible to lysis. A third phenotype (PNH II) was of intermediate sensitivity, about 3 to 5 times more susceptible than normal cells. Most patients have a mixture of type I and type III cells, but mosaics of type I, type II, and type III, as well as type I and type II, are also observed. The biochemical and molecular basis of the phenotypic mosaicism of PNH was elucidated by Holguin, respectively (see “ Membrane Inhibitor of Reactive Lysis ” and “Somatic Mutation of PIGA Underlies the Absence of GPI-AP in PNH”).
Elegant, rigorous in vitro studies of the aberrant interactions of complement with PNH erythrocytes indicated that two defects underlie the complement-mediated hemolysis that characterized the disease. One abnormality resulted in greater activity of the amplification C3 convertases of the classical and alternative pathways of complement, and the other caused PNH erythrocytes to be markedly more sensitive to the cytolytic activity of the membrane attack complex (MAC) of complement. The basis of these two complement regulatory abnormalities remained a mystery until the 1980s, when PNH erythrocytes were shown to be deficient in both CD55 and CD59 ( Figs. 14-2 , 14-3 ).
Paroxysmal Nocturnal Hemoglobinuria and Complement
The chronic intravascular hemolysis that is the hallmark clinical manifestation of PNH is mediated by the APC (see Fig. 14-3 ). The APC is a component of innate immunity. This ancient system evolved to protect the host against invasion by pathogenic microorganisms. Unlike the classical pathway of complement that is part of the system of acquired immunity and requires antibody for initiation of activation, the APC is in a state of continuous activation, armed at all times to protect the host. The APC cascade can be divided into two functional components: (1) the amplification C3 and C5 convertases and (2) the cytolytic MAC (see Fig. 14-3 ). The C3 and C5 convertases are enzymatic complexes that initiate and amplify the activity of the APC and ultimately generate the MAC (the MAC is the common cytolytic subunit of the classical and lectin pathways of complement as well as the APC).
Because the APC is primed for attack at all times, elaborate mechanisms for self-recognition and for protection of the host against APC-mediated injury have evolved. Both fluid-phase and membrane-bound proteins are involved in these processes. Normal human erythrocytes are protected against APC-mediated cytolysis primarily by CD55 and CD59 (see Fig. 14-3 ). These proteins act at different steps in the complement cascade. CD55 regulates the formation and stability of the C3 and C5 convertases, whereas CD59 blocks the formation of the MAC. Deficiency of CD55 and CD59 on the erythrocytes of PNH is the pathophysiologic basis of the direct antiglobulin test–negative, intravascular hemolysis that is the clinical hallmark of the disease.
Decay Accelerating Factor
In 1983, two groups reported that PNH erythrocytes were deficient in CD55. CD55, first identified by Hoffmann in 1969 and subsequently purified by Nicholson-Weller and colleagues in 1982, is a 70 kD protein that inhibits the formation and stability of the C3 convertases of complement. Thus, the absence of CD55 provided a plausible explanation for the greater binding of activated C3 to PNH erythrocytes that had been observed in in vitro experiments (see Fig. 14-3 ). Detailed studies, however, demonstrated that CD55 does not regulate the activity of the MAC of complement. Those results implied that PNH erythrocytes were deficient in a second complement regulatory protein that was functionally distinct from CD55.
Membrane Inhibitor of Reactive Lysis
In 1989, Holguin and colleagues reported the isolation from normal erythrocytes of an 18 kD protein called MIRL (CD59) that protected PNH red cells against complement-mediated lysis. As anticipated, PNH cells were found to be deficient in CD59, and additional studies by those investigators and others demonstrated that CD59 inhibits complement-mediated lysis by blocking the assembly of the MAC.
By comparing expression of CD55 and CD59 on PNH I, PNH II, and PNH III erythrocytes, the functional basis of the different complement-sensitivity phenotypes was determined. Those studies showed that PNH III cells are completely deficient in both CD55 and CD59, whereas PNH II cells are partially deficient in the two complement-regulatory proteins, and PNH I cells have normal expression. Thus the variability in sensitivity to lysis among the different phenotypes is explained by quantitative differences in expression of CD55 and CD59 ( Fig. 14-4 ). Additional experiments demonstrated that the combined deficiency of CD55 and CD59 was sufficient to explain the enhanced susceptibility of PNH erythrocytes to hemolysis in acidified serum.
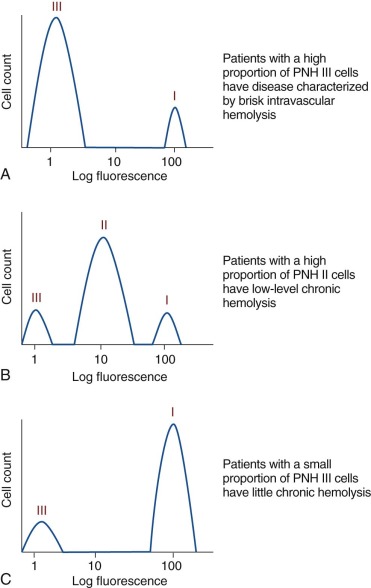
Inherited Mutations of CD55 and CD59
Of the two complement regulatory proteins, CD59 is more important than CD55 in protecting cells from complement-mediated lysis in vivo. Antigens of the Cromer-related blood group complex are located on CD55, and rare cases of a null phenotype called Inab have been reported. Like PNH cells, Inab erythrocytes are deficient in CD55, but unlike PNH erythrocytes, CD59 expression is normal on Inab red cells. Although Inab erythrocytes bind more activated C3 when exposed to acidified serum, they undergo little or no hemolysis. Further, subjects with the Inab phenotype have no known hematologic abnormalities, and in particular, they have no clinical evidence of hemolysis. These observations show that isolated deficiency of CD55 does not produce the PNH syndrome.
In contrast, a patient with an inherited, isolated deficiency of CD59 had a syndrome that was indistinguishable from PNH. Clinically the patient experienced recurrent episodes of hemoglobinuria, suggesting that CD59 is essential for protecting erythrocytes against complement-mediated lysis in vivo. Recurrent thromboembolic events were also observed in this patient. That patient had normal CD55 expression, but in vitro, his cells were susceptible to hemolysis in acidified serum, implying that CD59 deficiency accounts primarily for the positive Ham’s test in PNH.
More recently, Nevo and colleagues reported on five patients from four unrelated families, who presented in infancy with symmetric muscle weakness accompanied by hypotonia and absent deep tendon reflexes involving the legs more than the arms. The neurologic disease was classified as chronic inflammatory demyelinating polyneuropathy. Symptomatic episodes were accompanied by hemolytic anemia characterized by reticulocytosis, negative direct antiglobulin test, elevated serum lactate dehydrogenase (LDH) concentration, and low serum haptoglobin concentration. Leukopenia and anemia were not observed. There was no report of thrombotic complications, although the oldest of the patients in the study was 5 years old. Whole exome sequencing of DNA from one of the patients identified a homozygous missense mutation in the gene that encodes CD59. Subsequent studies identified the same mutation in the other four patients. The mutation segregated with the disease in the families and had a carrier rate of 1 per 66 among Jewish subjects of North-African origin. Cell surface expression of CD59 was absent from the erythrocytes of patients, while expression of CD55 was normal. Together, these studies confirm the importance of CD59 in the pathophysiology of the complement-mediated hemolysis of PNH and suggest an important pathologic role for aberrant regulation of the cytolytic MAC of complement in chronic inflammatory demyelinating polyneuropathy and perhaps other diseases characterized by loss of myelin and axonal integrity.
While the above studies provide interesting insights into the individual function of CD55 and CD59 in vivo, it is important to remember that, in PNH, both proteins (as well as all other GPI-APs on the abnormal hematopoietic clone) are deficient. Therefore it is the combined deficiency of CD55 and CD59 that results in the markedly abnormal susceptibility of the red cells of PNH to complement-mediated lysis.
Congenital Abnormalities of Genes Involved in Glycosyl Phosphatidylinositol–Anchored Protein Synthesis
Germline mutations in genes ( PIGN , PIGM , PIGO , PIGL , PIGV, and PIGA ) involved in biosynthesis of the GPI-anchor moiety have been reported. Notably, hemolysis and bone marrow failure are not observed in patients with germline mutation of GPI-anchor assembly proteins even in the case of germline mutant PIGA (see “ Epidemiology ”). The phenotype of patients with germline mutation of genes involved in GPI-AP assembly varies but often involves skeletal anomalies; neurologic abnormalities, including mental retardation and seizures; and hyperphosphatasia.
Paroxysmal Nocturnal Hemoglobinuria and Deficiency of Glycosyl Phosphatidylinositol–Anchored Protein
Beck and Valentine reported in 1951 that neutrophils from patients with PNH were deficient in leukocyte alkaline phosphatase. Thus leukocyte alkaline phosphatase was the first protein found to be deficient in PNH, but the first erythrocyte membrane protein found to be deficient was acetylcholinesterase. In 1959, Auditore and Hartmann presented evidence that the extent of the acetylcholinesterase deficiency correlated with the severity of the hemolysis. More detailed studies by others showed that PNH I red cells had a relatively normal amount of acetylcholinesterase, while PNH III erythrocytes were profoundly deficient. Although the deficiency of acetylcholinesterase plays no role in the abnormal susceptibility of PNH red cells to complement-mediated lysis, ultimately the observations that the red cells lack acetylcholinesterase and that the neutrophils lack alkaline phosphatase provided important insights into the fundamental defect that underlies PNH because both proteins were eventually shown to be GPI-anchored.
If only CD55 or only CD59 were deficient in PNH, it would have been logical to hypothesize that the disease was due to mutations affecting the gene that encodes the particular protein. That PNH cells were deficient in multiple proteins (CD59, CD55, and acetylcholinesterase on red cells and leukocyte alkaline phosphatase on neutrophils), however, eliminated (based on mathematic probability) the possibility that the gene for each protein was mutant. Rather, a more plausible hypothesis was that the PNH defect involved a posttranslational modification common to all of the proteins that are deficient in PNH. In 1984, Medof and colleagues reported that isolated CD55 spontaneously reincorporated into erythrocyte membranes. This property of CD55 had been appreciated by Hoffmann in 1969. Working with the butanol-saturated aqueous phase of a crude extract prepared from normal human erythrocyte stroma, Hoffmann showed that the sample contained a factor capable of inhibiting complement-mediated lysis by accelerating the decay of the C3 convertase. He further showed that this substance (that he called DAF [CD55] of stroma [DAF-S]) had the capacity to reincorporate into red cells and remain functionally active. In 1980, Low and Zilversmit demonstrated that alkaline phosphatase that had been solubilized from cells by butanol extraction exhibited the capacity to bind to phospholipid vesicles. In this case the incorporation was thought to be due to the attachment of a phosphatidylinositol moiety to the enzyme. Subsequent experiments demonstrated that isolated acetylcholinesterase also spontaneously reincorporated into cell membranes. Previous studies had shown that alkaline phosphatase, acetylcholinesterase, and 5′-ectonucleotidase (another protein that is deficient in PNH) were released from the cell surface by treatment with phosphatidylinositol-specific phospholipase C (PIPLC) (see Fig. 14-1 ). The cumulative work of a number of investigators showed that both the capacity to reincorporate into lipid membranes and susceptibility to cleavage by PIPLC was characteristic of a group of amphipathic membrane proteins that shared the common structural feature of being anchored to the cell surface through a GPI moiety (see Fig. 14-1 ). The structural link between CD55 and acetylcholinesterase, alkaline phosphatase, and 5′-ectonucleotidase (CD73) was made in 1986 by Davitz and colleagues and Medof and colleagues when those investigators presented evidence that CD55 is a GPI-AP. Subsequent studies confirmed that CD59 is also a GPI-AP. The results of those studies suggested the following paradigm: All proteins that are deficient in PNH are GPI-anchored, and all GPI-APs that are expressed by normal hematopoietic cells are deficient in PNH (see Fig. 14-2 ). All data to date are consistent with this postulate.
GPI-APs are functionally diverse. In addition to the complement regulatory proteins (CD55 and CD59) and enzymes (acetylcholinesterase, alkaline phosphatase, and 5′-ectonucleotidase [CD73]) discussed above, proteins with a variety of receptor, adhesion, and immune modulatory functions (e.g., FcγRIIIb [CD16b], urokinase plasminogen activator receptor [CD87], endotoxin binding protein receptor [CD14], and LFA-3 [CD58]) are also GPI-anchored. Further a number of proteins whose function is unknown are GPI-anchored. Over 80 eukaryotic proteins have been shown to be GPI-anchored, and approximately 30 of these are found in humans. Currently, the list of proteins deficient in PNH numbers at least 23 (see Box 14-1 ). This number is less than the total number of GPI-anchored human proteins because PNH is an acquired disease that affects only hematopoietic cells. Thus GPI-APs that are present on somatic tissues other than hematopoietic cells are expressed normally in patients with PNH. Despite the fact that PNH cells lack a number of functionally diverse membrane constituents, the only pathologic component of the disease that is unequivocally causally related to GPI-AP deficiency is the abnormal susceptibility of the erythrocytes to complement-mediated lysis due to deficiency of CD55 and CD59.
The GPI anchor is a complex structure with at least 25 proteins being essential for assembly of the moiety (see Fig. 14-1 ). Hypothetically the PNH phenotype would result if any of these proteins were nonfunctional because if the GPI-anchor is not synthesized, GPI-APs are not expressed. Accordingly, it seemed probable that PNH would be found to be heterogeneous at the molecular level because mutations affecting any one of several genes that encode elements critical for GPI-anchor assembly would produce the disease phenotype. In 1992 and 1993, however, two groups published the surprising finding that GPI-protein deficient lymphocyte cell lines derived from different patients with PNH all belonged to the same complementation class (Class A) of GPI-AP–negative cell lines. Additional experiments confirmed that the PNH cell lines had the same biochemical defect as the complementation Class A mutants. Like the Class A mutants, the PNH cell lines failed to synthesize N-acetylglucosaminyl-phosphatidylinositol, the first intermediate in the pathway of GPI-anchor assembly (see Fig. 14-2 ).
Somatic mutation of PIGA underlies the absence of GPI-AP in PNH. A gene that restores normal expression of GPI-APs in the complementation Class A PNH cell lines was identified by Kinoshita and colleagues in 1993. As predicted by the studies cited above, the gene, called PIGA (for phosphatidylinositol glycan, class A), encodes a protein that is essential for the normal synthesis of GlcNAc-PI (see Fig. 14-2 ). Subsequently, Takeda and colleagues showed that PIGA complements the deficient expression of GPI-APs in PNH lymphoblastoid cell lines and that those cell lines harbored somatic mutations in PIGA. Together those studies defined both the biochemical and the molecular basis of the deficiency of GPI-APs in PNH.
Additionally Takeda and colleagues observed that a heterozygous mutation was sufficient to produce the PNH phenotype. As presaged by the studies of Hyman and colleagues, the dominant expression of the somatic mutation was explained when PIGA was mapped to chromosome Xp22.1. Because males have a single X chromosome, any functionally significant mutation affecting PIGA is expressed. Females are functionally haploid due to X-inactivation. Therefore, somatic mutations in PIGA appear dominant when they occur on the active X chromosome (see Fig. 14-2 ).
Analysis of PIGA has revealed that the same mutation can be identified in isolated neutrophils, monocytes, and lymphocytes from individual patients with PNH, confirming that the disease involves the hematopoietic stem cell. More than 150 PIGA mutations have been identified in affected cells of patients with PNH. Only a few large deletions have been observed. The remaining mutations consist of nucleotide substitutions of the missense or nonsense types, or small deletions or insertions that cause frame-shifts and introduce premature termination codons. The mutations are distributed randomly over the entire coding region and at splice junctions. Although some mutations have been observed in more than one patient, most are unique, indicating absence of mutational hot spots in PIGA . These observations suggest that clonal selection depends on complete or nearly complete inactivation of PIGA such that expression of GPI-APs is below a critical threshold. Therefore all mutations in PNH cause loss of function (partial or complete) of PIGA .
Studies of PIGA mutations have also provided insights into the molecular basis of the phenotypic mosaicism of PNH. Cloned lymphocyte cell lines were established from the peripheral blood of a patient whose erythrocytes were a mixture of PNH I, PNH II, and PNH III cells. Based on expression of GPI-APs, lymphocyte clones with four different phenotypes were observed. Analysis of clones with normal expression of GPI-AP revealed no somatic PIGA mutations. In contrast, between lymphocyte clones with two distinct phenotypes based on the pattern of GPI-AP expression (i.e., PNH II and PNH III), four discrete PIGA mutations were identified. In the lymphocyte clones with the PNH II phenotype, a missense mutation that changed a highly conserved amino acid was found. This finding suggests that cells with partial expression of GPI-APs (PNH II) are derived from stem cells with mutations that incompletely inactivate PIGA. In the case of the lymphocyte clones with the PNH III phenotype, three separate mutations were identified, each of which was expected to inactivate completely the PIGA gene product. Collectively these experiments demonstrate that the phenotypic mosaicism that is characteristic of PNH is a consequence of genotypic mosaicism. Further, because any mutation that completely inactivates PIGA results in PNH III cells, phenotypically identical cells can have different PIGA genotypes.
Studies of the pattern of X chromosomal inactivation indicated that, in the female patient with four different PIGA mutations, the abnormal clones were not derived from a common ancestor. Further, that each of the mutations was discrete demonstrated that the mutational events occurred independently rather than by clonal evolution. These results demonstrate that PNH is not strictly a monoclonal process and have important implications for the origins of the disease.
Studies of mutational frequency in PNH have produced conflicting results. Some of the disparity is almost certainly due to differences in experimental design and interpretation of data. While an abnormally high mutational rate could contribute to generation of multiple PIGA mutant clones, invoking such a process is not necessary to explain the oligoclonality observed in some patients with PNH. Rather, a growth/survival advantage for any PIGA -mutant GPI-AP− stem cells likely accounts for the outgrowth of multiple PNH clones. In this sense PNH can be viewed as an example of Darwinian evolution occurring in the bone marrow. According to this hypothesis, hematopoietic stem cells with a randomly acquired mutant PIGA exist in the marrow in a quiescent state and become apparent only when deficiency of one or more GPI-APs bestows upon those mutant cells a proliferative or survival advantage. Putatively the selection pressure that is applied to the marrow, such as immune attack, involves one or more GPI-APs such that hematopoietic stem cells with mutant PIGA that lack expression of GPI-APs have a survival advantage.
What is the basis of clonal selection and clonal expansion? Despite the progress that has been made in determining the basis of the abnormal sensitivity of the erythrocytes to complement-mediated lysis and the global absence of GPI-APs from hematopoietic cells, an issue that is fundamental to a more complete understanding of PNH remains largely enigmatic. In order for PNH to become clinically evident, the hematopoietic stem cells bearing the mutant PIGA must expand so that progeny sufficient to produce symptoms and signs of the disease are generated. In many instances GPI-AP− cells dominate hematopoiesis in patients with PNH, suggesting that the mutant stem cell has a greater proliferative capacity, a survival advantage relative to GPI-AP–sufficient (GPI-AP+) stem cells, or both. That PIGA mutations are necessary for the development of PNH is incontrovertible. At issue is whether PIGA mutations are both necessary and sufficient to account for the PNH syndrome and whether the PIGA mutation provides an absolute or a conditional growth/survival advantage.
PNH differs from monoclonal hematopoietic stem cell disorders such as CML, in which the t(9;21) that generates the fusion protein BCR–ABL is sufficient to account for the proliferative advantage of the mutant cell and in which all normal hematopoiesis is progressively and invariably displaced as a consequence of the uncontrolled proliferation of a transformed clone. In PNH the peripheral blood is a mosaic of normal and abnormal cells and the proportion of GPI-AP+ to GPI-AP− cells varies greatly among patients. Further, that ratio tends to remain fixed over long periods of observations. PNH is, therefore, more aptly characterized as a nonmalignant, clonal process, but the basis of the clonal selection and clonal expansion are incompletely understood.
The oligoclonal nature of PNH suggests that a powerful selection process that is most likely based on phenotype is at work in the bone marrow. According to this hypothesis, stem cells with mutant PIGA have an advantage because of some pathologic process (perhaps immune mediated) that involves a GPI-AP. For example an autoimmune process could arise in which the target antigen is a GPI-AP expressed on hematopoietic stem cells. Under those circumstances PIGA mutant stem cells (lacking GPI-APs) would escape immune-mediated destruction because the target antigen is absent.
Hematopoietic cells express a relatively large number of functionally diverse GPI-APs (see Box 14-1 ). Thus absence of all GPI-APs is probably not required for the clonal selection of the mutant stem cells. Rather the selective advantage may be dependent on the absence of a single protein that is GPI-anchored, and the reason for the global deficiency of GPI-AP is that PIGA is located on the X chromosome. According to this supposition, an autosomal gene encodes the GPI-AP that is conditionally detrimental (e.g., an antigen targeted for immune destruction or a receptor for a negative growth regulator). Inasmuch as two alleles rather than one must be mutated, the probability of inactivating an autosomal gene through somatic mutagenesis is remote compared to the probability of inactivating an X-linked gene. Therefore stem cells with a deficiency of the detrimental GPI-AP are most likely to arise as a consequence of PIGA mutations, as is the case in patients with chronic lymphocytic leukemia treated with Campath1H (anti-CD52). Assuming that the GPI-AP complement regulatory proteins are not the targets of the underlying pathologic process, the hemolytic anemia that is the clinical hallmark of PNH may represent an epiphenomenon that is a consequence of the chromosomal location of PIGA .
Although a compelling case can be made in support of the paradigm that the PIGA mutation bestows a conditional survival advantage upon the affected stem cell, the hypothesis that the mutant stem cells have an absolute growth advantage must also be considered. Data that both support and challenge this hypothesis can be found. The most compelling argument against an intrinsic growth or survival advantage for PIGA mutant cells is made by the results of studies using transgenic mice. By using homologous recombination, Kawagoe and colleagues disrupted Piga (the murine homologue of PIGA ). Only mice with a low degree of chimerism survived. Among those animals, the percentage of GPI-AP− erythrocytes ranged from about 1% to 5%. During 10 months of observation, the ratio of GPI-AP− to GPI-AP+ peripheral blood cells did not increase. Studies by others using conditional knockout technology have confirmed these observations. Together these observations indicate that the PIGA mutation does not confer an absolute growth or survival advantage.
What Is the Origin of the PIGA-Mutant Stem Cells? Patients with PNH often have more than one PIGA mutant clone. A tenet of the Darwinian selection hypothesis as it applies to PNH is that PIGA mutant hematopoietic stem cells are present during the time when the selection pressure is applied to the bone marrow. PIGA -mutant hematopoietic elements have been identified in the peripheral blood and bone marrow of normal volunteers, providing experimental support for this concept, although whether the GPI-AP− cells identified in a normal individual originate from hematopoietic stem cells with mutant PIGA has been questioned. While there are experimental data that suggests that the mutational frequency of the gene is abnormally high, that PIGA is located on the X chromosome (so that only one allele need be mutated for the phenotype to become apparent) likely accounts primarily for the existence of multiple discrete clones in many patients with PNH.
A Two-Step Model of Paroxysmal Nocturnal Hemoglobinuria Pathogenesis
Studies by Inoue and colleagues suggest that a two-step mechanism may account for the unique pathophysiology of PNH. Those investigators identified two patients whose PIGA -mutant cells had a concurrent, acquired rearrangement of chromosome 12. Detailed analysis showed that in both cases, the chromosome 12 rearrangement resulted in disruption of the 3′ untranslated region (3′UTR) of HMGA2 . As a consequence, a negative regulatory region of the locus was disrupted, resulting in ectopic expression of the gene. HMGA2 is a member of the high mobility group of proteins (HMGA1a, HMGA1b, HMGA2) that function as architectural transcription factors. HMG members possess no intrinsic transcriptional activity. Instead these nonhistone phosphoproteins orchestrate assembly of stereospecific transcriptional regulatory proteins into enhanceosomes. The cellular targets of HMGA2 are incompletely defined but appear to include cyclin A and E2F1.
Molecular studies established a causal role for HMGA2 in benign mesenchymal tumors. Rearrangement of 12q13-15 is observed in these neoplasms, but tumorigenesis does not depend on generation of chimeric proteins derived from fusion of HMGA2 with specific translocation partners. Rather, clonal expansion induced by HMGA2 appears to result from deregulated expression of the protein. For the two PNH patients, ectopic expression was a consequence of deregulation of expression caused by truncation of the 3′UTR-containing elements that are the target of microRNA let-7a that negatively regulates HMGA2 expression. Additional studies will be required to determine whether aberrant expression of HMGA2 underlies clonal expansion in PNH patients without structural abnormalities of 12q13-15, but studies by Murakami and colleagues support this hypothesis.
Recently, Sugimori and colleagues reported concurrent mutation of JAK2 ( JAK2V617F ) in three patients with PNH. This mutation drives clonal expansion in myeloproliferative disorders including polycythemia vera, primary myelofibrosis, and essential thrombocytosis. Thus the JAK2V617F mutation likely contributes to clonal expansion of hematopoiesis in this subgroup of patients with PNH.
In contrast to a disorder characterized by cellular transformation and malignant cellular growth, PNH manifests many of the characteristics of a benign tumor because there is limited expansion of PIGA mutant clones (the peripheral blood of patients is a relatively stable mosaic of normal and abnormal cells), PIGA mutant cells respect tissue boundaries (there is no invasion of nonhematopoietic tissues), PIGA mutant cells respond appropriately to signals that normally regulate hematopoiesis (function is not autonomous), and transformation into acute leukemia occurs rarely (PNH is not a premalignant condition). The studies of Inoue and colleagues suggest the concept of PNH as a benign tumor of the bone marrow with aberrant expression of HMGA2 acting in concert with mutant PIGA (and the consequent deficiency of GPI-APs) to produce the proliferative phenotype that underlies clonal expansion. These studies did not define whether (or if) the aberrant expression of HMGA2 works additively or synergistically with mutant PIGA to produce the proliferative phenotype.
The findings of Inoue and colleagues provide new insights into the etiology of the nonmalignant clonal hematopoiesis of PNH. These studies support a two-step process consisting of (1) clonal selection based on phenotype (i.e., GPI-AP deficiency resulting from mutant PIGA ) and (2) clonal expansion as a consequence of a second somatic mutation that bestows an additional growth/survival advantage. Clonal selection may induce exit of PIGA mutant stem cells from a dormant state, thereby favoring acquisition of the second mutation that underlies clonal expansion. The variable expansion of PIGA -mutant clones among patients and among mutant clones in the same patient could be explained by heterogeneity involving the second genetic event that partners with mutant PIGA . According to this hypothesis, mutant PIGA is promiscuous with respect to the second mutant gene with which it partners. But the benign nature of PNH suggests that genes involved in clonal expansion of PIGA mutant stem cells are different from those that underlie malignant clonal diseases such as acute leukemia. In fact, concurrent mutation of PIGA with mutation of genes that drive tumorigenesis may be synthetically lethal, accounting for the low incidence of transformation of PNH into a malignant clonal process such as acute leukemia.
In summary, the basis of the clonal dominance in PNH is incompletely understood, however, available evidence supports a two step-model of pathogenesis ( Fig. 14-5 ). Step 1 of this model is clonal selection. In this case, a loss-of-function mutation affecting PIGA causes deficiency of GPI-APs on affected stem cells. These mutant stem cells have a relative survival advantage in the setting of immune-mediated bone marrow failure (e.g., AA) (see Fig. 14-5 ). Clonal expansion, step 2 of the PNH pathogenesis model, is envisioned as a consequence of clonal evolution in which mutation of a second gene, working in concert with the consequences of mutant PIGA , bestows upon the double mutant stem cell an absolute proliferative/survival advantage (see Fig. 14-5 ). The extent of expansion of a particular mutant clone and the dominance of one PIGA mutant clone in the presence of other PIGA mutants may be mediated by the nature of the second event that may be molecularly heterogeneous (see Fig. 14-5 ). Alternatively, a limited number of second mutations may partner with mutant PIGA, and the extent of clonal expansion may be determined by how the second mutation affects gene function.
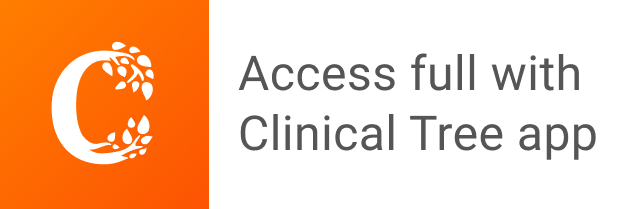