Ophthalmology in Aerospace Medicine
Thomas J. Tredici
Douglas J. Ivan
And God said, “Let there be light.” Genesis 1:3
Vision has always held a dominant place in the attributes necessary for flying. This was recognized by early pioneers, such as Drs. William Wilmer and Conrad Berens, who established the first laboratory to study the visual problems of the flyer in 1918 at the Air Service Medical Research Laboratory at Mineola, Long Island, New York (1). This almost total dependence on vision is evident now, as astronauts have reported the necessity of vision for orientation in space. Vision occurs peripherally at the eye and centrally in the brain. In the eye, the retina receives electromagnetic energy (photons) and, through a photochemical reaction, converts it into electrical signals. The nervous impulses are relayed to the occipital area of the brain where the signals are processed and interpreted as vision.
APPLIED ANATOMY AND PHYSIOLOGY OF THE EYE
The human eyes are protected by bony orbits that are shaped like a quadrilateral pyramid, thereby allowing good exposure of the cornea anteriorly to facilitate vision. Posterior openings in the orbits allow cranial nerves and blood vessels from the brain to communicate with the eye. Six extraocular muscles rotate each eye in all directions of the visual field.
Globe
The globe measures approximately 25 mm in diameter and is composed of three coats. The two outer layers, the scleral and uveal coats, are involved with support, protection, and nutrition. The inner coat, the retina, contains the light sensitive elements. The clear cornea has a shorter radius (7.5 mm) than the sclera (12.0 mm) (Figure 14-1).
As shown in (Figure 14-2) the normal eye is a +60 D refractive system, +45 D supplied by the cornea and +15 D by the unaccommodated lens. Therefore, we see that small changes in the radius of curvature of the cornea can cause substantial changes in refraction. This can be accomplished by the use of contact lenses, (orthokeratology) or surgical procedures such as radial keratotomy (RK), photorefractive keratectomy (PRK), or laser in situ keratomileusis (LASIK), as well as other corneal refractive surgical (CRS) procedures.
The uvea lies inside the scleral coat, it is the pigmented, vascular portion of the eye consisting of the choroid posteriorly, and the ciliary body and iris anteriorly. Aqueous humor is produced by diffusion and secretion in the epithelium of the ciliary processes. The ciliary muscle, innervated by the parasympathetic nervous system, supplies the contractile force necessary to change the shape of the lens during accommodation. The pupil is the opening in the iris. Its size is controlled by a delicate balance of the sympathetic and parasympathetic tone of the autonomic nervous system, principally in response to the level of illumination.
The intraocular lens lies just behind the iris, held in place by zonular fibers that are inserted into the lens capsule and the ciliary processes on the ciliary body (Figure 14-1). In order to see clearly at near, one must increase the power of the lens; this is known as accommodation. The parasympathetic impulse to the ciliary muscle constricts the circular part of the muscle, the zonular fibers then slacken, and the elastic capsule of the lens makes it more spherical thereby increasing its dioptric power. Accommodation decreases with age; by the mid forties, most aviators will be presbyopic and need spectacles to view clearly the panel, charts, and radios. Also of importance is that the eye is in focus for monochromatic yellow only, being hypermetropic for red and myopic for blue (2).
The retina is the innermost photosensitive layer. The neurosensory retina consists of ten layers. The light-sensitive elements are the rods and cones (Figure 14-3). The rods serve vision at low levels of illumination (scotopic vision) whereas the cones are effective both for medium and high levels of illumination (mesopic and photopic vision) and for color vision. The cones are mainly concentrated in the fovea centralis where the density has been measured at 47,000 cones/mm2. The optic disc or blind spot is located
15 degrees nasal to the fovea and covers an area of 7 degrees in height and 5 degrees in width (Figure 14-4).
15 degrees nasal to the fovea and covers an area of 7 degrees in height and 5 degrees in width (Figure 14-4).
The vitreous, a clear colorless gel-like structure, fills the posterior four fifths of the globe. The vitreous is 99.6% water. The complaint of vitreous floaters is universal and usually innocuous. Floaters are usually due to the collapse of the protein/collagen scaffolding, thereby thickening and casting a shadow on the retina. More ominous floaters, which should be investigated at once, are often referred to as a shower of floaters; these are probably red blood cells. A dark floating membrane should be investigated for the possibility of a retinal detachment.
Adnexa
The adnexa of the eye are the extraocular muscles, the eyelids, and the lacrimal apparatus (Figure 14-5). There are six extraocular muscles attached to each globe, and because of the strong desire for fusion and the maintenance of single binocular vision both foveas are directed on the object of regard by both reflex and voluntary action. The lacrimal gland is located in a bony fossa on the frontal bone. It secretes the aqueous portion of the precorneal tear film. The corneal epithelium is covered by a three-layered film composed of an outer oily layer derived from the meibomian glands of the tarsal plate, the middle aqueous layer from the lacrimal gland, and the inner mucoid layer that arises from the goblet cells of the conjunctiva. The drainage system for tears consists of a small punctum or opening in the innermost edge of the upper and lower lids near the caruncle. These openings lead into a common canaliculus then into the lacrimal sac, exiting under the inferior turbinate in the nose.
The eyelids provide protection for the cornea, blinking six to eight times per minute; this blink also enhances the optical qualities of the cornea. The eyelids are closed by action of the
orbicularis oculi muscles, which are innervated by the facial nerve (cranial nerve VII), and are opened by the levator palpebrae superioris muscles, innervated by the oculomotor nerve (cranial nerve III), elevation of the lids is assisted by Müller’s muscle innervated by the sympathetic nervous system. Reduction of sympathetic tone to Müller’s muscle can bring on the droopy eyelids seen on long, exhausting missions.
orbicularis oculi muscles, which are innervated by the facial nerve (cranial nerve VII), and are opened by the levator palpebrae superioris muscles, innervated by the oculomotor nerve (cranial nerve III), elevation of the lids is assisted by Müller’s muscle innervated by the sympathetic nervous system. Reduction of sympathetic tone to Müller’s muscle can bring on the droopy eyelids seen on long, exhausting missions.
![]() FIGURE 14-2 The normal values for the optical properties of the eye. RAD, radius; D, diopter; n, index of refraction. |
VISUAL PRINCIPLES
Vision is essential in all phases of flying and is most important in the identification of distant objects and in perceiving details of shape and color. The visual sense also allows the judgment of distances and gauging of movements in the visual field. In flying modern aircraft and spacecraft, near vision is also exceedingly important because it is absolutely necessary to be able to read the instrument panel, radio dials, charts, visual displays, and maps. At night, although one’s vision is reduced, one must still rely on vision to safely fly the aircraft.
Physical Stimuli
The electromagnetic spectrum extends from the extremely short cosmic rays with wavelengths on the order of 10−16 m to the long radiowaves several kilometers in length (Figure 14-6). The part of the spectrum that stimulates the retina is known as visible light and extends from 380 nm (violet) to approximately 760 nm (red). A nanometer is a millionth of a millimeter, or 1 × 10−9 m. Adjacent portions of the spectrum, although not visible, affect the eye and are, therefore, of interest. Wavelengths of 380 nm and shorter, down to 180 nm, are known as ultraviolet or abiotic rays. Exposure of the eyes to this portion of the electromagnetic spectrum produces ocular tissue damage; the severity of the damage depends on the intensity and duration of exposure. Wavelengths longer than 760 nm, up to the microwaves, are known as infrared (IR) or heat rays. These rays, too, may cause ocular tissue damage, depending on the intensity and exposure time. The light intensity in extraterrestrial space above 30,000 m is approximately 13,600 footcandle (ft-c). At 3,000 m on a clear day, the light intensity is approximately 12,000 ft-c and approximately 10,000 ft-c at sea level. Water vapor, dust particles, and air in the atmosphere absorb some of the sun’s light; in addition, selective absorption occurs. Ultraviolet light shorter than 200 nm is absorbed by dissociated oxygen. Ultraviolet light 200 to 300 nm is absorbed by the ozone layers in the atmosphere; this is fortunate because wavelengths 200 to 300 nm are the most damaging to the eye. These wavelengths produce the actinic keratoconjunctivitis that welders have when they fail to wear protective lenses. These wavelengths of 200 to 300 nm are no problem until an altitude of approximately 40,000 m is reached. This is approximately the height of the second ozone layer. Above this altitude, these ultraviolet wavelengths must be considered. Recent work done in the space program shows that the most abiotic rays have a wavelength of 270 nm (3). They must be filtered by protective visors, or they will severely limit the time that can be spent in extravehicular space activities. The rays that reach the earth, therefore, are 300 to 2,100 nm in wavelength, with an intensity varying between 10,000 ft-c at ground level to approximately 13,000 ft-c at presently attainable altitudes.
Visual Functions
The visual apparatus, stimulated by light, must primarily perform three basic functions. It must be able to perceive an object by the detection of light emitted or reflected from it; this is known as light discrimination. Second, it must be able to perceive the details of an object; this is known as visual acuity. Third, it must allow one to judge distances from objects and to perceive movement in the field of vision. These latter two functions combined are known as spatial discrimination. Obviously, all of these functions are perceived simultaneously; however, in this chapter, they will be discussed separately. Light discrimination consists of brightness sensitivity, which is the ability to detect a dim light; brightness discrimination, which is the ability to detect a change or difference in the brightness of light sources; and color discrimination, which is the ability to detect colors. As noted in Figure 14-7, when the illumination is below a certain intensity, approximately 10−6 log mL, the eye does not respond and there is total darkness. As the level of illumination increases, one begins to see shapes and objects; this is rod or scotopic vision. At best, this vision is on the order of 20/200 to 20/400 in scope. As illumination increases, such as with snow in full moonlight of 10−2 log mL, the threshold for the cones is reached, and this is known as mesopic vision; here, both rods and cones are functioning. A further increase in illumination (such as with white paper under 100 ft-c, equivalent to approximately 102 log mL, causes the cones alone to be functional; this is known as photopic vision. The cones are now sensitive to color, and minute details can be appreciated. Increasing the illumination beyond 102 log mL does little to enhance visual efficiency. The upper limit of tolerance for normal vision is 104 to 105 log mL of luminance. This would be equivalent to staring at the sun or at the detonation of a nuclear weapon. The eye can adapt to this tremendous range of illumination because of the dual system of rods and cones in the retina. The rods contain the photosensitive pigment rhodopsin and are sensitive to minute quantities of light energy. They are also sensitive to motion but not to color. The cones contain photosensitive pigments with maximum
absorption at 445 nm (blue), 535 nm (green), and 570 nm (red). The cones must have much more light energy than the rods to be stimulated; however, the cones can perceive fine detail and discriminate colors.
absorption at 445 nm (blue), 535 nm (green), and 570 nm (red). The cones must have much more light energy than the rods to be stimulated; however, the cones can perceive fine detail and discriminate colors.
The three psychologic components to color are hue, saturation, and brightness. Hue is the component denoted by naming a color, such as red, yellow, or orange. This is closely related to the wavelength of the light. Saturation refers to adding white light to the pure color so as to decrease the saturation of this color. For instance, a spectral red becomes pink when it is mixed with white light. The hue is still red, but its saturation has now been decreased. Finally, brightness relates to the amount of luminous flux reaching the eye. In essence, a source of high intensity or luminance seems brightly colored, for example, bright red or bright yellow, whereas a source of low intensity or luminance appears dark or dull colored (4).
At night or under low levels of illumination, the fovea, containing all cones, becomes a relative blind spot. Therefore, best vision is attained at night by looking 15 to 20 degrees off-center to utilize the part of the retina containing both cones and rods. As is noted in the dark adaptation curve (Figure 14.11), the cones adapt quickly, taking 6 to 8 minutes; however, the rods are much slower in adapting, requiring another 20 to 30 minutes in the dark. Rods and cones also have different peak sensitivities. The relative luminosity curves of photopic and scotopic vision show that scotopic vision (rod function) peaks at 510 nm, whereas photopic vision (cone function) peaks at 555 nm, as shown in Figure 14-8. The difference in these peak sensitivities is the basis of the Purkinje shift. The luminosity curves also show why red filter goggles with a cutoff at 610 nm allow the cones to receive enough light for the individual to function, while greatly reducing the light to the rods and allowing dark adaptation to take place.
![]() FIGURE 14-9 Rod and cone density in the retina. (Adapted from Chapanis RN. Vision in military aviation. Technical Report 58-399. Wright Field: Wright Aeronautical Development Center, 1958.) |
The second of the basic functions, visual acuity, is the ability to see small objects, to distinguish separate details, or to detect changing contours. This is usually measured in terms of the reciprocal of the visual angle subtended by the detail. Central (foveal) visual acuity is high, whereas peripheral visual acuity is poor, less than 20/200. The retinal distribution pattern of rods and cones causes this difference in visual acuity, as shown in Figure 14-9. The cones are dense in the foveal and macular areas and even have a 1:1 nerve fiber-to-brain relationship in the fovea, whereas images outside of the macular area lose detail, becoming worse in the peripheral retina. In certain areas of the peripheral retina, many hundreds of rods may be connected to a single nerve fiber. This is an excellent system for picking up a minimum of light energy or detecting motion but poor for perceiving detail. Visual acuity is influenced by the refractive state of the eye. Visual acuity can be separated into four basic types: minimum visible, which is the ability to see a point source of light, with intensity determining whether it can be seen or not; minimum perceptible, which is the ability to see small objects against a plain background, where size (the angle subtended) and contrast become the determining factors; minimum separable, which
is the ability to see objects as separate when close together (also known as two-point discrimination); and minimum distinguishable, which is the form sense, usually measured on the Landolt C or Snellen charts and resolving 1 minute of arc break in the C or thickness in letters at 20/20 visual acuity.
is the ability to see objects as separate when close together (also known as two-point discrimination); and minimum distinguishable, which is the form sense, usually measured on the Landolt C or Snellen charts and resolving 1 minute of arc break in the C or thickness in letters at 20/20 visual acuity.
Another form of testing visual resolving power is by the use of contrast sensitivity and gratings (Figure 14-10). The most useful form for visual testing is the sine form. The use of this sinusoidal form allows the ready application of a powerful mathematical tool, the Fourier transform. A sine grating of 30 cycles/degree visual angle may be compared with 1 minute of visual angle or 20/20 Snellen equivalent. A contrast sensitivity plot shows that the human visual system is most sensitive in the area of 2 to 4 cycles/degree (5). This method of testing may show reduced contrast sensitivity in conditions such as amblyopia, multiple sclerosis, optic neuritis, cataract, and possibly glaucoma.
Presently, this testing procedure has more value in the examination and evaluation of aircrew with ocular disease, unexplained visual loss, and research purposes (6).
The third important visual function necessary for aerospace flight is depth perception. This is the judging of distance and the perception of motion in the visual field. Distance judgment, or depth perception, is the ability to judge absolute distance or, more commonly, the relative distance of two or more objects. It is aided by conscious and subconscious cues learned from experience, such as aerial perspective, relative motion, relative size, distribution of light and shadow, overlapping contours, and, perhaps the most important of these monocular factors, motion parallax.
The binocular factors of convergence and stereopsis are also involved in this process. Stereopsis, resulting from the disparity of images on the retina of the two eyes, is the most important factor in judging the distance of near objects. In flying aircraft, however, maximum practical limit of stereopsis is believed to be only 200 m (600 ft).
Vision is a complex physiologic and psychological process that necessitates a decoding or interpretation of signals coming from the sensor (the eye) to the brain. Environmental stresses may disrupt the delicate physiologic balance necessary for maintaining clear vision and are discussed in ensuing sections.
VISION IN THE AEROSPACE ENVIRONMENT
The aviator and astronaut function in a hostile environment. In this section, the effects of this environment on the eye and vision are discussed. Some of the factors affecting vision include hypoxia, decompression, glare, high-speed acceleration, and, if one were to proceed into space, excessive electromagnetic energy, zero gravity, and other factors. All of these factors can degrade vision and, therefore, one’s ability to perform duties at the most effective level possible.
Environment and the Eye
Hypoxia
Vision is the first of the special senses to be altered by a lack of oxygen, as evidenced by diminished night vision. The extraocular muscles become weakened and incoordinated and the range of accommodation is decreased, causing blurring of near vision and difficulty in carrying out near visual tasks. From sea level to 3,000 m is known as the indifferent zone because ordinary daytime vision is unaffected up to this altitude; however, slight impairment of night vision occurs such that all combat crews flying at night should use oxygen equipment from the ground up. From 3,000 to 5,000 m is the zone of adaptation. Some impairment of visual function occurs; however, this impairment can be overcome sufficiently for duties to be performed. At this altitude, retinal vessels become darker and cyanotic, arterioles show a compensatory increase of 10% to 20% in diameter, retinal blood volume increases up to four times, retinal arteriolar pressure, along with systemic blood pressure, increases slightly, the pupil constricts, and, at 5,000 m, a loss of approximately 40% in night vision occurs. Accommodation and convergence decrease, and one’s ability to overcome heterophorias decreases. All of these changes can return to normal when the flyer returns to ground level or uses oxygen. Physiologic compensatory reactions enable flyers to perform normal tasks unless they remain at this altitude
for long periods without oxygen. The zone of inadequate compensation is 5,000 to 8,000 m, so-called because the physiologic processes can no longer compensate for the lack of oxygen. The visual disturbance described above becomes more severe, with reaction time and response to visual stimuli becoming sluggish. Heterophorias can no longer be compensated for and become heterotropias with double vision. Accommodation and convergence are so weakened as to cause blurred vision and diplopia. Night vision is most seriously impaired. Once again, if one were not subjected to too long a stay at this altitude, all changes would be reversed by the use of oxygen or a return to sea level. Above 8,000 m is the zone of decompensation, or lethal altitude. Circulatory collapse occurs, with loss of vision and consciousness, and permanent damage to the retina and/or brain may result from the lack of circulation and hypoxia. Commercial aircraft and other aircraft with pressurized cabins maintain cabinequivalent pressures of lower or equal to 2,500 m pressure altitude. None of the aforementioned visual effects is felt at this altitude except for an almost immeasurable effect on night vision. For smokers, the altitude zones can be considerably lower due to the effects of carbon monoxide (7).
for long periods without oxygen. The zone of inadequate compensation is 5,000 to 8,000 m, so-called because the physiologic processes can no longer compensate for the lack of oxygen. The visual disturbance described above becomes more severe, with reaction time and response to visual stimuli becoming sluggish. Heterophorias can no longer be compensated for and become heterotropias with double vision. Accommodation and convergence are so weakened as to cause blurred vision and diplopia. Night vision is most seriously impaired. Once again, if one were not subjected to too long a stay at this altitude, all changes would be reversed by the use of oxygen or a return to sea level. Above 8,000 m is the zone of decompensation, or lethal altitude. Circulatory collapse occurs, with loss of vision and consciousness, and permanent damage to the retina and/or brain may result from the lack of circulation and hypoxia. Commercial aircraft and other aircraft with pressurized cabins maintain cabinequivalent pressures of lower or equal to 2,500 m pressure altitude. None of the aforementioned visual effects is felt at this altitude except for an almost immeasurable effect on night vision. For smokers, the altitude zones can be considerably lower due to the effects of carbon monoxide (7).
Reduced Barometric Pressure
Decompression sickness is a disturbance that affects the flyer as a result of reduced barometric pressure. Infrequently with decompression sickness, a transitory visual defect consisting of homonymous scotoma or even hemianopia may occur, followed by headache that closely resembles migraine. Even more rarely, the aviator may be afflicted by transitory hemiplegia, monoplegia, aphasia, and disorientation. In rare cases, permanent visual impairment occurs. See Chapter 3 for further detail and discussion of decompression sickness.
Visual Environment
The aviator’s visual environment is constantly changing. One travels from night to day, from sunlight to shadow, from well-structured scenes to empty visual fields. Fortunately, the eye is quite adaptable, functioning in light levels from 1 × 10−6 log mL to 1 × 105 log mL. For example, the brightness of the full sun on a cloud is approximately 6 × 103 log mL, snow in full moonlight is 1 × 10−2 log mL, and snow in starlight is 1 × 10−4 log mL. As higher altitudes are attained, the sky darkens, being lighter at the horizon and darker at the zenith. This reverses what is considered normal light distribution, creating a bright view below and darkness above. At high altitudes, less haze is evident and the sun’s rays are much more intense, so that 13,600 ft-c of illumination occurs at 30,000 m. A higher proportion of ultraviolet rays are also found at this altitude. For each kilometer (3,300 ft) increase in altitude, ultraviolet radiation increases by approximately 6%. Glass sunglass lenses decrease the intensity of light and protect against ultraviolet radiation as well. Plastic spectacle lenses must have attenuators in the plastic to filter out ultraviolet radiation. Newer materials, however, such as polycarbonate, being used in the windscreens of modern aircraft substantially reduce the amount of ultraviolet radiation that enters the cockpit. This material cuts off most of the ultraviolet light below 380 nm. The aviator’s vision is also affected by the lack of detail in the sky at altitude. This empty field, or space myopia, causes a decrement in his visual capabilities. Finally, changes occur in the appearance of sunlight and areas of shadow. Areas in shadow are illuminated by scattered light, but less light scatter and brighter sunlight occur at high altitude, so that the contrast between the sunlit and shadowed areas increases.
Visibility
Much of modern flying is done in the cockpit. This necessitates good near vision and is dependent on having an adequate amount of visual accommodation. In spite of instruments and radar scopes, one must still see outside the cockpit to land and take off, fly formation, navigate, and, especially, watch for other aircraft. Multiple related factors allow the aviator to see objects in the environment (1): the size of the target, which is relative to its distance (2); the luminance or overall brightness (3); the degree of retinal adaptation (4); the brightness and color contrast between the target and background (5); the position of the target in the visual field (6); the focus of the eye (7); the length of time the object is seen; and (8) atmospheric attenuation.
The visibility of an object depends mostly on its size and contrast with the background. In daylight, with the best of contrast (a black object on a white background), an object would be seen at near the threshold of visual acuity, subtending 0.5 minute of arc, or the equivalent of 20/10 (Snellen) vision. A speck of light against a black background, such as a star, can be seen even when it is much smaller and obviously at enormous distances; however, this example is not a function of visual acuity but only of light perception. A star appears bigger because it is brighter not because it subtends a larger visual angle. The visibility of objects is lost as the contrast is reduced between the object and its background. In such a case, the object, now with lower contrast, must be much larger or nearer before it can be seen. In conditions of haze or mist, such a marked loss of contrast exists that even a large object may not be seen at all. Testing techniques, such as contrast sensitivity function tests, hold promise for the identification of individuals whose systems function more effectively at lower contrast thresholds. The visibility factors outlined in the preceding text are, to a certain extent, interrelated, so that a reduction in one may be compensated for by an increase in one of the others. For instance, an object may be so small or so far away that it is just below the threshold of visibility. It may be made visible by an increased illumination or by improving the contrast between it and its background or both. In other instances, the object may be better perceived when more time is spent viewing it.
Targets in the periphery of the visual field must be proportionally larger to be seen. To get maximum visibility, the target will have to be seen within 1 degree of fixation (fovea). When the object in the peripheral field is moving, it is easier to detect.
One final factor capable of degrading target acquisition is empty field or space myopia. Older theories explained that the resting state of the eye was one of zero accommodation. More sophisticated testing techniques (laser optometer) show that in some individuals, the resting state of the eye is actually one in which a small amount of accommodation is exerted, thereby defocusing the eye for distance vision. In the so-called resting states, these individuals have 0.75 to 1.00 D of myopia thereby degrading their distance visual acuity because their resting focus is 1 to 1.5 m distant from the eye. This is said to occur in both emmetropic and myopic individuals. Moderately farsighted individuals (hypermetropes), however, may find that this accommodative tonus is actually advantageous and that their distance vision perhaps may be enhanced. In bright daylight, the small pupil produced compensates somewhat for the space myopia by increasing the depth of field; however, a better method of overcoming this induced myopia is to fix on a distant object. Actually, anything more distant than 15 to 18 m helps to relax the accommodation sufficiently to improve the distance visual acuity. Night myopia, which is similar to empty field and space myopia, is discussed in the following section on night vision.
Night (Scotopic) Vision
Night vision is extremely important in aviation. It is quite different from day (photopic) vision. The eyes must be used differently at night for the aviator to gain maximum usefulness of vision. The aviator must understand the principles of night vision and must practice using the eyes at night to gain efficient vision at night.
Not all parts of the retina are alike in their reaction to light. A small, central area containing only cones is responsible for maximum visual acuity and for color discrimination, but it fails to operate under low intensities of illumination. This is the fovea, the area with which one reads and where one focuses objects in the direct line of vision. It gives us central vision, which is useful in high and moderate illumination (photopic and mesopic conditions).
In the remaining peripheral area, both the rod-type and cone-type receptors are present. The peripheral retina is capable of less acute visual perception and of only poor color determination, but it functions under low illumination or scotopic conditions. According to the widely accepted duplicity theory of vision, the human eye is an eye within an eye. Central vision requires light of approximately 1 × 10−3 log mL intensity or greater. Bright moonlight gives approximately 1 × 10−2 log mL. Hence, in light that is less intense than moonlight, little central vision is evident. Peripheral vision requires only one-thousandth as great an intensity 1 × 10−6 log mL or more. On a dark, starlit night, the individual sees only with the peripheral area of the retina. This explains why pilots often complain that they are able to see an aircraft at night only to have it disappear when they look directly at it. To keep an object in sight at night, one must learn to look off to the side at approximately a 15- to 20-degree angle. When the light intensity is between 1 × 10−3 and 1 × 10 degrees log mL, both the rods and cones are functioning, and mesopic vision occurs (Figure 14-7).
Individuals can determine which type of vision they are using by noting whether they have color sense. The cones perceive all colors. Rods pick up colors only as shades of gray. Most of the cones are in the central area of the retina, so that if colors were recognized at night, one would have central vision; however, if everything were to appear in shades of gray, one would only have peripheral or rod vision.
Dark adaptation is the process by which the eye adjusts for maximum efficiency in low illumination. It is commonly experienced when one first enters a theater or walks into darkness from a brightly lit room. The central area of the retina dark-adapts in approximately 6 to 8 minutes, but this part of the retina is useless for night vision. The peripheral area dark-adapts in approximately 20 to 30 minutes, although further slight adaptation continues over a period of 2 days (Figure 14-11). This peripheral area is not sensitive to dark-red light (630 nm or longer in wavelength). Such light is not perceived even as gray, so dark adaptation occurs in the periphery in dark-red light as though no light existed. This characteristic is fortunate because, by wearing red, lighttight goggles before a flight, pilots can read or rest in a brightly lit room while the peripheral areas of their retinas are dark-adapting.
Dark adaptation is an independent process in each eye. It is slow to develop in the dark and is quickly lost in the light. The aircrew must be so familiar with the location of their equipment and controls that lights are unnecessary for making adjustments in flight. The aviator should avoid gazing at exhaust stacks or any other bright light sources. When using light at night in the plane, such as in reading instruments, maps, or charts, as little light as possible should be employed and for as brief a time as possible, and red light should be used; however, red lighting does create problems, such as accommodative fatigue and reduction of color perception. Therefore, red light is no longer favored for cockpit visual activities. When an individual who is exposed to bright light closes one eye, the closed eye remains darkadapted, although the exposed retina of the open eye has been bleached.
Dark adaptation also depends on an adequate supply of vitamin A in the diet. Vitamin A is found in vegetables that are green or were green at some stage of development, such as lettuce, carrots, cabbage, peaches, tomatoes, green peas, and bananas. Other sources of vitamin A include milk, eggs, butter, cheese, and liver. A deficient diet or an illness that decreases the vitamin A supply impairs night vision and a return to normal vision may take several months, even when large doses of vitamin A are ingested. Excessive vitamin A ingestion, such as taking in large doses of vitamin capsules, is worthless to a normal person. Various drugs have also been studied and have not been found to improve a normal person’s dark adaptation.
Without supplemental oxygen, the average percentage decrease in night vision capability is 5% at 1,100 m altitude, 18% at 2,800 m, 35% at 4,000 m, and a 50% decrease in night vision capability at 5,000 m altitude. Lack of oxygen, fatigue, and excessive smoking all reduce the ability to see well at night. For military flying, oxygen should be used from the time of takeoff for maximum visual acuity. Fatigue should be prevented, insofar as possible, by obtaining adequate sleep before flying. Hypoxia resulting from carbon monoxide poisoning affects brightness discrimination and dark adaptation in the same way as altitude-induced hypoxia. As an example, 5% saturation with carbon monoxide has the same effect as flying at 3,000 m without oxygen. Smoking three cigarettes before a flight may cause a carbon monoxide saturation of 4%, with an effect on visual sensitivity equal to an altitude of 2,800 m or a 15% to 18% decrease in night vision.
During World War II, much work was done on the use of red cockpit illumination. The use of a red light having a wavelength greater than 630 nm illuminating the cockpit is desirable from the viewpoint of dark adaptation. The intent was to retain the greatest rod sensitivity possible while permitting an effective illumination for foveal vision; however, with the increasing use of electronic devices for navigation, the importance of the pilot’s visual efficiency inside the cockpit has increased markedly. Therefore, low-intensity white cockpit lighting is presently advocated because it affords a more natural visual environment within the aircraft without degrading the color of objects that are not self-luminous. The disadvantage of the previously used red lighting caused red markings on aerial maps to be invisible when viewed in this light. Red light also tends to create or worsen near-point blur in prepresbyopic, presbyopic, and, at times, hypermetropic pilots. Because of the chromatic aberration of the eye, humans are hypermetropic for red (8).
Ultraviolet light has been used for cockpit illumination and has a disconcerting side effect if it were to become reflected directly into the eye. These radiations produce a fluorescence of the crystalline lens in the eye, giving the pilot a sensation that he is flying in a fog. Properly adjusting the ultraviolet lamps and reducing their intensity can overcome this fluorescence problem to some degree. Radiations from these lamps are not injurious to the eyes because, even at highest intensity, they are still far below the threshold for affecting the corneal epithelium.
During World War II, the problem of night vision was studied intensively by numerous scientists, but no single, satisfactory test of night vision was developed. The United States Air Force (USAF) did develop the radium plaque night-vision tester, which is a self-illuminating Landolt C target; however, because it contains radium, it is rarely used now (9).
At present, the best test of night vision is the Goldmann-Weekers Dark Adaptometer. This instrument is capable of determining the dark-adaptation curve of an individual with great detail and accuracy. It is obviously not something that should be done on everyone because it is time-consuming, the apparatus is expensive, and is available only in research institutions and larger clinics. With this instrument, one can establish the threshold of night vision in an individual. The testing results in the familiar dark-adaptation curve (Figure 14-11).
NIGHT-VISION GOGGLES
Modern technology has also introduced night-vision goggles (NVGs), which enhance vision at night over and above that possible by the naked eye (Figure 14-12). Currently available NVGs can intensify ambient light to approximately 1,000 times (×1,000). Several electro-optical devices are available to improve vision at night, including NVG and forwardlooking infrared (FLIR) systems. Most NVG systems are helmet mounted and look like binoculars. To make objects and landscape visible at night, NVGs usually employ two image-intensifier tubes to amplify or intensify low levels of reflected and emitted ambient light. Image-intensifier tubes are sensitive to some visible and short-wave IR radiation, but a minimum amount of ambient light is needed to excite the green phosphor screen and produce visible images.
The NVG-intensified image resembles a black and white television image except that it is in shades of green instead of in shades of gray, due to the selected display phosphor.
The image that is seen by the aircrew member is not a direct view, but an image displayed on a phosphor screen. The NVG system is analogous to using a microphone, amplifier, and speaker to amplify a faint sound and make it audible. In both cases, some of the “natural fidelity” may be lost in the amplification process.
The image that is seen by the aircrew member is not a direct view, but an image displayed on a phosphor screen. The NVG system is analogous to using a microphone, amplifier, and speaker to amplify a faint sound and make it audible. In both cases, some of the “natural fidelity” may be lost in the amplification process.
NVGs enhance night vision over unaided scotopic vision; however, they do have significant limitations. The performance limitations include visual acuity of approximately 20/40 at best, a field of view of 40 degrees or less, degraded depth perception, little or no stereopsis, and a different spectral sensitivity than the human eye. Therefore, training and experience with NVGs is critically important for flying safety.
A FLIR device consists of a cockpit-or helmet-mounted video monitor that displays picture from an internal IR sensor that is usually fixed forward (i.e., slaved to the nose of the airplane). These sensors are sensitive to the long wavelength IR (thermal) and provide excellent resolution. IR sensors can detect radiation in either the 3,000 to 5,000 nm or the 8,000 to 12,000 nm spectral range. A FLIR must have thermal radiation available, but many objects radiate measurable amounts of IR energy in the spectral range.
Electro-optical Design
A brief description of the operating principles of NVGs will make it easier to understand their workings and limitations. Ambient light entering the intensifier tubes is focused by an objective lens onto a photocathode. The schematic diagram of an intensifier tube is presented in Figure 14-13. When photons of ambient light strike the photocathode, which is sensitive to visible and near-IR radiation, electrons are released, creating a cascading effect. The number of electrons released from the photocathode is proportional to the number of photons striking it. The electrons are then accelerated and multiplied by a microchannel plate, which acts like a large array of photomicromultiplier tubes. The microchannel plate, approximately the size of a nickel, guides the accelerated electrons to a phosphor screen that produces an intensified light image. The light amplification is referred to as the gain of the device. The gain is the ratio of the light delivered to the eye by the phosphor screen to the light striking the objective lens. Modern NVGs have a gain of 400 to 1,000.
NVGs do not turn night into day. Although pilots are usually impressed the first time they look through NVGs, many complaints occur the first time they fly with them. Although it is true that visual function with NVGs is impressively enhanced over scotopic function in many ways, NVG performance is inferior compared to normal photopic function. The degradation in visual performance that NVGs impose must be emphasized to aircrew.
Much more detailed information on NVGs, operational issues, environmental considerations, and fitting techniques is explained in the publication, Night Vision Manual for the Flight Surgeon by Miller and Tredici (10).
SPATIAL DISCRIMINATION, STEREOPSIS, AND DEPTH PERCEPTION
In aviation, it is important to accurately localize in three-dimensional space. When this cannot be done, one becomes spatially disoriented, which is a marked hazard to the flyer. Under +1 Gz acceleration, one orients to the earth by proprioceptive impulses from various parts of the body, from receptors in the semicircular canals and vestibular apparatus, and with the strongest cue to orientation, the visual system. Linear and angular accelerations are capable of producing spatial disorientation, especially when outside visual reference is excluded; however, when adequate external visual references are available, spatial disorientation usually does not occur. The pilot’s ability to resist spatial disorientation, then, is greatly enhanced by adequate visual references and is diminished by mental stress. The visual cues to the perception of depth are both monocular and binocular. The monocular cues are learned, and some investigators believe that they can be improved by study and training. These cues, however, are the ones that can be the most easily tricked by illusions. Conversely, stereopsis, which is the most important binocular cue, is innate and inescapable. When flyers have this capability, it remains with them, even when the learned
cues are sparse, such as at night, under conditions of low visibility, and in unfamiliar surroundings. Unfortunately for flyers, however, the maximum range at which their stereoscopic vision is useful is only up to 200 m. This is not to imply that stereopsis is required in flying an aircraft because numerous individuals who lack stereopsis still make good aviators; however, when the pilot does have stereopsis, so much the better. Therefore, stereoscopic testing procedures should be retained in the flight examination. The stereoscopic test for flying is probably the single most revealing component of the visual examination. Individuals who pass the stereoscopic test down to 15 to 20 seconds of arc must, of necessity, have well-functioning visual systems. They must have two eyes that are equally balanced: visual acuities must be excellent to attain this kind of arc disparity, they must have normal retinal correspondence, and their motility status must be functioning normally in at least the straight-ahead position. In essence, even if stereopsis had nothing to do with flying, retaining the stereopsis portion of the ophthalmologic examination is wise.
cues are sparse, such as at night, under conditions of low visibility, and in unfamiliar surroundings. Unfortunately for flyers, however, the maximum range at which their stereoscopic vision is useful is only up to 200 m. This is not to imply that stereopsis is required in flying an aircraft because numerous individuals who lack stereopsis still make good aviators; however, when the pilot does have stereopsis, so much the better. Therefore, stereoscopic testing procedures should be retained in the flight examination. The stereoscopic test for flying is probably the single most revealing component of the visual examination. Individuals who pass the stereoscopic test down to 15 to 20 seconds of arc must, of necessity, have well-functioning visual systems. They must have two eyes that are equally balanced: visual acuities must be excellent to attain this kind of arc disparity, they must have normal retinal correspondence, and their motility status must be functioning normally in at least the straight-ahead position. In essence, even if stereopsis had nothing to do with flying, retaining the stereopsis portion of the ophthalmologic examination is wise.
Depth Perception (Spatial Localization)
Depth perception is the mental projection onto visual space of a perceived object in real space. Correlation of the real object in real space with that projected in visual space results in accurate depth perception. Both monocular and binocular cues to depth perception exist.
The monocular cues are as follows:
Size of the retinal image (size constancy)—being able to judge the known and comparative size of objects is an important cue
Motion parallax—the relative speed of motion of images across the retina; objects nearer than fixation move against the observer’s motion, distant objects move in the same direction as the observer’s motion
Interposition—one object obscured from vision by another
Texture or gradient—detail loss at increasing distances
Linear perspective—parallel lines converging at distance
Apparent foreshortening—for example, a circle appears as an ellipse at an angle
Illumination perspective—light sources are usually assumed to be from above
Aerial perspective—distant objects appear more bluish and hazy than do near objects
The binocular cues are as follows:
Convergence—the value of this cue is questionable and is generally used only for near distances
Accommodation—also useful only for near distances
Stereopsis—this is the visual appreciation of three dimensions during binocular vision, occurring during fusion of signals from slightly disparate retinal points, which are disparate enough to stimulate stereopsis but not so disparate as to cause diplopia
The two most important monocular factors for flying are considered to be motion parallax and size of the retinal images. All monocular cues are derived from experience and are subject to interpretation. Stereopsis is believed to be the most important binocular cue and is based on a physiologic process that is innate and inescapable. Like visual acuity, stereopsis can be graded and is known as stereo acuity, which is measured in seconds of arc of disparity. Owing to the limiting factors such as the interpupillary distance, stereopsis is not reliable beyond 200 m (600 ft) (11).
Stereopsis (one element in the perception of depth) is measured by several different instruments. One can measure stereopsis for near distances, on the Verhoeff depthperception apparatus, where stereo acuity is measured at 1 m without special optical devices. Stereopsis for near distances also can be measured by the Wirt (Titmus) circles. In this case, the eyes are dissociated with polarizing lenses. Stereoscopic vision for distance is measured in testing devices, such as the Bausch and Lomb Ortho-Rater, Titmus, Keystone, or Optec instruments. Using these instruments, separate images are presented to each eye, and lenses in the instruments project the images to infinity. In essence, these are tests of stereoscopic vision for distance, and many examiners are not aware that in some motility disturbances, such as microstrabismus, the candidate may have normal stereoscopic vision (depth perception) for near but not for distance or vice versa.
COLOR VISION
Aeromedical experts and flyers have emphasized the importance of normal color vision since World War I. In 1920, Drs. William H. Wilmer and Conrad Berens noted in their article, The Eye in Aviation, that the proper recognition of colors played an important part in the success of aviators (1). In modern aviation, both civil and military, color discrimination requirements have not diminished, but rather have expanded dramatically. For instance, aviators and aircrew must be able to identify assorted colored light signals and navigation lights, as well as the colors of various reflecting surfaces such as ground targets, flags, smoke, and flares. It is important to be able to identify colors used on maps and charts even in less than optimal lighting and, especially in the military, for ascertaining subtle color differences in targets and terrain. Modern aircraft now incorporate full spectral color in electronic flight information systems (EFIS) designed to speed up flight information to aircrew primarily because color increases efficiency without demanding further conscious effort. Under certain conditions, such as bright daylight, the color contrast of these displays decreases. With hypoxia and impoverished visual conditions, such as fog, smoke, haze, or in dim light, color perception degrades, with color defectives disproportionately degrading compared to normals under decreasing illumination, hypoxia, and fatigue (12, 13, 14, 15, 16, 17, 18, 19). Chronic hypoxia at cabin altitudes between 8,000 to 10,000 ft above ground level (AGL), where supplemental oxygen has traditionally not been supplied, has emerged as an issue of concern, particularly in military
operations. All of these factors place additional emphasis on an aviator having normal color vision than in the past.
operations. All of these factors place additional emphasis on an aviator having normal color vision than in the past.
Color vision deficiencies can be congenital, acquired, or induced artificially. Congenital color deficiencies are almost always red/green and are much more common in males, whereas blue/yellow defects affect both sexes equally and are rarely congenital, rather a result of ocular disease or toxicity. Congenital red/green defects are inherited as a sex-linked recessive trait. Approximately 10% of all males versus 0.5% of females are congenitally red/green color defective (20,21). Unlike sex-linked red/green color vision deficiencies, congenital blue/yellow defects are extremely rare, occurring equally in 0.001% to 0.007% of males and females (22,23). Acquired defects typically first present as blue/yellow deficiencies and are estimated to be present in 5% to 15% of the general population (24, 25, 26, 27, 28). As a result, identification of true color normalcy requires testing for red/green and blue/yellow defects, both congenital and acquired.
How much color deficiency is required for safe and effective flying remains under heavy debate, particularly in civil aviation. In July 2002, the National Transportation and Safety Board (NTSB) identified defective color vision as a contributing factor in the crash of a commercial aircraft in Tallahassee, Florida (29). As a consequence of that mishap, the NTSB recommended that the Federal Aviation Administration (FAA) reevaluate existing color vision requirements and adopt more effective testing methodologies. Consequently, there is no question that this accident and the proliferation of color in the modern cockpit mandates a fresh look at this relationship. For example, color defectives make more mistakes, take more time, and need to be closer to color-based targets when compared to color normals.
For most purposes, individuals with normal color vision can be effectively screened using pseudoisochromatic plates (PIPs). Few color normals will fail properly administrated PIP tests, whereas color abnormals will have great difficulty scoring like a normal, unless they have learned techniques to defeat these tests. In many cases, however, subjects with mild color vision deficits perform as poorly as those with deficiencies that are more significant. Lanterns, long used as secondary occupational tests since the 1800s, have fallen out of favor because of relative unavailability, lack of operational relevance in the modern environment, and other technical problems. For example, the color threshold test (CTT) developed at the Army Air Forces School of Aviation Medicine in World War II permitted mild color defectives to enter aviation training; however, the scores used for the CTT were based on field studies involving the ability to distinguish at the time between colored pyrotechnic flares, the biscuit gun, and wire coding schemes (30, 31, 32, 33). It was eventually abandoned once replacement Wratten filters were no longer made. The U.S. Navy developed the Farnsworth lantern, or FALANT, during World War II for naval signalmen (34,35). It was designed to qualify 20% of color defectives, presumably mild defectives believed to be safe in the signal environment at the time, into Navy career fields that required “normal” color vision; however, it no longer remains linked to current operational requirements. Furthermore, recent studies have shown it to pass much more severe defects and its utility to reliably identify color normals for modern applications has been challenged, especially by the NTSB (36, 37, 38, 39, 40, 41, 42, 43, 44, 45, 46, 47). Consequently, the USAF no longer uses it for color vision testing. In addition to assorted PIP tests, other tests of color vision such as Farnsworth’s Dichotomous Test (Panel D-15) and FM-100 tests have been used for aviation. Many of these are effective in identifying dichromat subjects, but become unreliable in testing less severe color vision defectives. None is as effective as an anomaloscope based on Rayleigh and Moreland metameric equations. Recently devised tests based on spatiotemporal luminance masking, such as the color assessment and diagnosis (CAD) test, or those employing isolated cone-specific contrast principles, such as the cone color test (CCT), may even displace PIPs and anomaloscopes as more convenient and definitive screeners (48, 49, 50, 51, 52, 53). Until then, the definitive gold standard color vision test against which all other color vision tests are compared is the anomaloscope (Table 14-1). The North Atlantic Treaty Organization (NATO)’s Working Group 24 (WG 24) recommended that color vision testing for modern aviation should involve an initial PIP test followed by an anomaloscope, if more definitive assessment is required (54). WG 24 also recommended abandoning use of all occupational lantern tests. However, all color vision tests that rely on reflected light, whether PIPs (Ishihara, Dvorine, AO, Hardy-Rand-Rittler, Igaku-Shoin, etc.) or hue discrimination tests (D15, Lanthony, FM100, etc.) must be presented with light of proper Kelvin temperature (Illuminant C). The most commonly used Illuminant C is the MacBeth light, with a temperature of approximately 6,000°K (13). All of these tests must also be properly administered, for example, given monocularly away from other light sources and applicable time limitations.
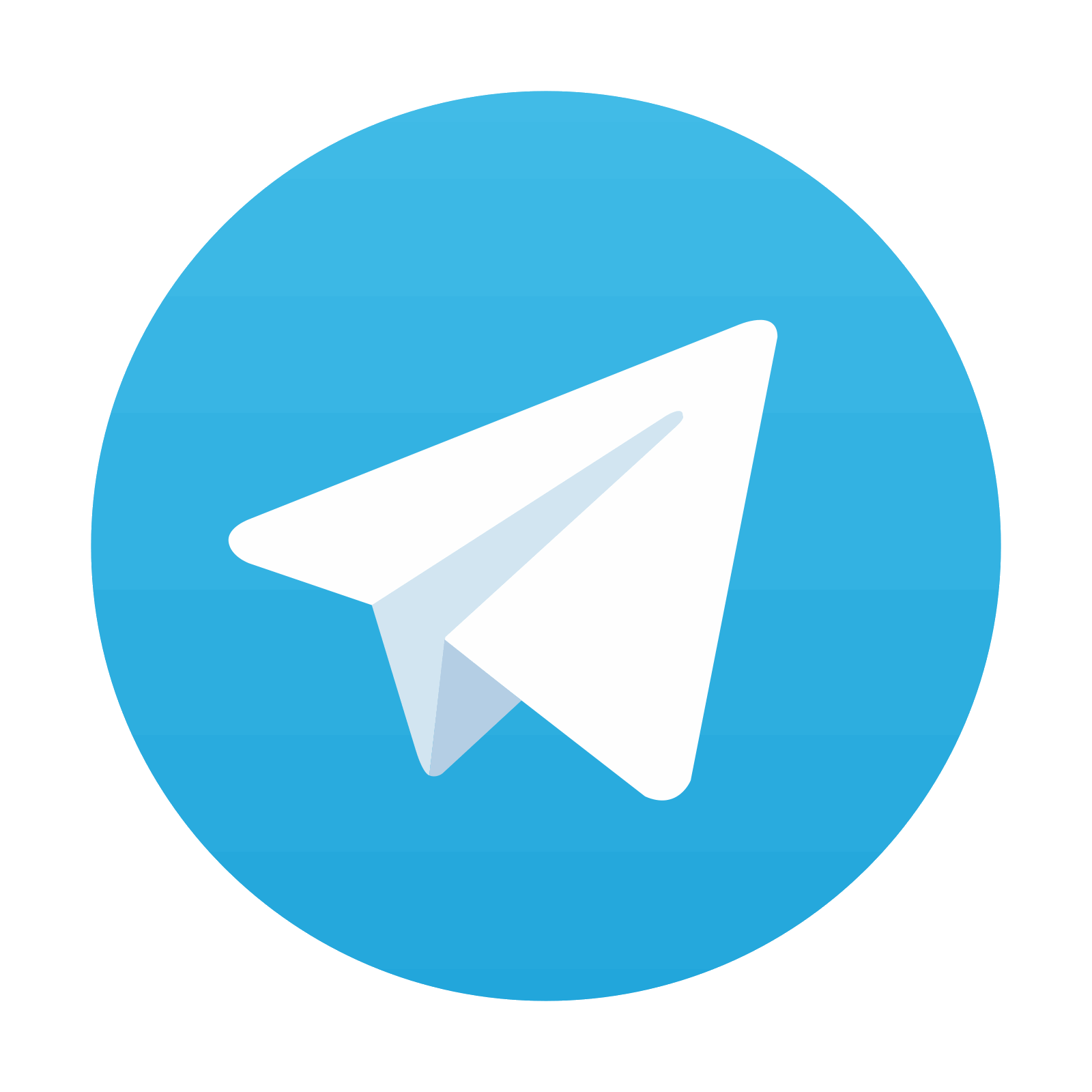
Stay updated, free articles. Join our Telegram channel
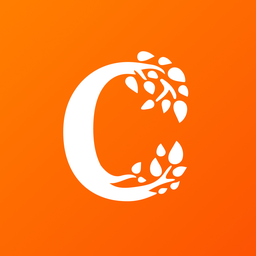
Full access? Get Clinical Tree
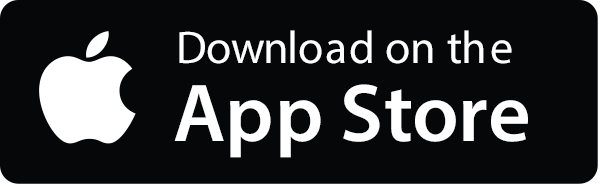
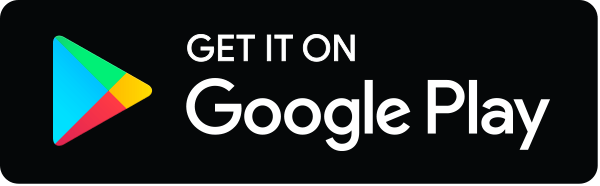