Dietary choices impact disease. In the outpatient setting, dietary choices influence our risk for heart disease, cancers, obesity, diabetes, and stroke1,2; poor dietary choices increase the chance for developing these chronic and acute conditions, many of which are among the leading causes of morbidity and mortality in the United States and the world.3,4 Five of the 10 leading causes of death in the United States are directly associated with dietary factors (heart disease, cancer, stroke, diabetes, and atherosclerosis).5 And dietary interventions can be a primary treatment for these conditions, with outcomes equivalent to or better than drug therapy.6
In the inpatient, hospital-based setting, a patient’s nutritional status also impacts clinical outcomes. This notion was first reported in surgical patients in the year 1936, in a study demonstrating that patients with baseline malnutrition undergoing peptic ulcer surgery had mortality rates of 33% compared to 3.5% in well-nourished controls.7 This pattern has been reported repeatedly since then, with preoperative malnutrition leading to increased morbidity and mortality in the surgical and trauma literature. With the prevalence of baseline malnutrition among hospitalized medical and surgical patients as high as 40%,8,9 many patients are at high-risk for poor outcomes and complications.
Anticipating and addressing a hospitalized patient’s dietary needs can therefore substantially impact their disease as well as their outcome. Simply put, providing adequate nutrition is essential to high-quality patient care. Given the extreme physiologic, metabolic, and homeostatic changes that can occur after severe injury, nowhere in medicine is this more true than in trauma, surgery, and critical care.
This chapter is about nutrition, the inflammatory stress responses to injury, and the nutritional needs of the polytrauma patient, for both macro- and micronutrients. The first major section “Nutritional Support” provides a thorough, up-to-date guide on nutrition: first, nutritional needs and requirements are addressed, in both the healthy patient as well as the critically ill, hypermetabolic trauma patient; second, we will outline the specifics of nutritional intervention, including both enteral and parenteral nutritional support; lastly, we cover some special patient populations. The second major section of this chapter, “Electrolyte Management,” is divided into the management strategies of four major electrolytes and their associated abnormalities—sodium and disorders of water balance, potassium, phosphorus, and magnesium.
Ensuring a patient meets their nutritional needs is vitally important: it is as fundamental to good outcomes as attending to their cardiac function, respiratory status, venous thromboembolism risk, and infection concerns. Addressing the energy and nutrient requirements in the multiple-injured trauma patient requires knowing the needs of a patient in good health as well as knowing how the post-injury inflammatory stress response can impact those needs.
This section is divided into two parts. The first part discusses nutrient utilization and requirements in a state of normal health (and thus trauma patients with only minor injuries), including a thorough investigation of all macronutrients and micronutrients. The second part reviews nutrition in abnormal states of health, particularly in the most severely injured trauma patients, and how their hypermetabolic, hypercatabolic, hyperdynamic inflammatory stress response impacts nutritional needs.
Normal health implies homeostasis, when there is balance among the metabolic regulatory mechanisms that act to keep the body in a smooth, continuous condition of regular physiologic function. Part of homeostasis involves ensuring that the body has adequate energy reserves as well as other nutrients to maintain this physiologic normalcy.10 In this section we discuss nutrient utilization and requirements in a state of normal health, and provide a conceptual framework underlying the basis and terminology of nutritional needs support. We will cover the three principle classes of food—namely proteins, carbohydrates, and fats (collectively the macronutrients)—as well as the other fundamental compounds without which humans could not survive: water and the micronutrients (vitamins and minerals). See Box 60-1.
BOX 60-1: The Confusing Language of Food Energy
There are two widely accepted units of measuring and quantifying food energy:
The International System of Units (SI, aka the “modern” metric system), which is the official system of measurement in almost every country in the world, measures energy in joules (J).13,14 Within this system food-related quantities of energy are typically expressed in kilojoules (kJ).
The “old” metric system measures energy in calories; as a unit of energy, it officially is the upper-case Calorie, or equally kilocalorie (kcal): 1 kcal (aka 1 Calorie) is equal to 1000 calories. The scientific definition of 1 kilocalorie is the amount of energy needed to raise the temperature of 1 kilogram of water 1°C from 14.5° to 15.5° at 1 atm. A conversion from the old to the new (SI) metric system is: 1 kcal = 1 Cal = 4.184 kJ.
The terminology is confusing. When speaking of nutrition and food energy, it is common to use the term “calorie,” with an implied meaning of kilocalories. For example, in the United States, the daily recommended value (DRV) for an average adult female is 2000 calories per day; this more accurately should be written 2000 kcal (2000 kcal = 2000 Calories). Therefore, it is technically incorrect to use calorie interchangeably with kilocalorie; the calories counted by clinicians and dieticians for nutritional purposes are really kilocalories.15
To make it even more confusing, the standard units used for food energy vary by region of the world, depending on a country’s adoption of the SI system. In some parts of the world, including the United States, food labels use the older system, and list Calories or kcals even though, as noted above, the Food and Drug Administration’s (FDA) Food Labeling Guide drops the “kilo-” and simply refers to food energy incorrectly as “calories.”16 In most other parts of the world food energy is expressed in SI unit of kilojoules. In the European Union food labels list both kJ (kilojoules) and kcal (kilocalories).17
In nutrition circles, including the Committee on Nomenclature of the International Union of Nutritional Sciences,18 it has been proposed that the SI unit of the kilojoule replace the old metric unit of kilocalorie as the unit of choice for defining the energy value of food. Such a change would align the nomenclature of food sciences with that of most other sciences, which use SI units and the metric system. In this chapter, however, we do not adopt the modern metric system, rather we use the language common in the United States: the kilocalorie.
Humans need energy to live: to do physical work; to maintain body temperature and concentration gradients; to transport, synthesize, degrade, and replace molecules which make up our body’s tissues.11 This energy is generated by the oxidation of various organic substances consumed in the diet, primarily the macronutrients: carbohydrates, fats, and proteins. The energy produced in the human body by the oxidation of macronutrients is the same as the heat of combustion of these substances in a lab. The major difference is that the body’s oxidation is carried out through multiple steps, allowing humans to capture the energy in an intermediate chemical form.
A human’s daily energy requirement allows for maintenance of metabolism and activity. This requirement is the level of energy intake from food that will balance energy expenditures. While the word “energy” is often used repeatedly in a discussion of nutrition, its use in this sense is overly general and nonspecific. “Energy” can refer to food energy, basal energy expenditure, potential energy, energy density, or many other aspects of nutrition. As it relates to what we eat, food energy (as measured in kilocalories or kilojoules) specifically refers the energy derived from protein, carbohydrates (aka sugars), and fat.
In addition to energy requirements, humans also have nutrient requirements, and therefore need protein (for essential amino acids), fats (for essential fatty acids), water, vitamins, and minerals to survive. Macronutrients and micronutrients, along with water, build the basis of nutrient utilization and needs. Each will be reviewed here:
Energy = Calories = kilocalories
A patient’s daily kilocaloric needs (aka total energy requirement) are often referred to as their total energy expenditure (TEE); strictly speaking, this is not completely accurate, as the energy needs (aka requirements) are determined by the energy expenditure. A better way to think about this is to appreciate that energy requirements are based on estimates of energy expenditure.10
The basal metabolic rate (BMR) reflects the energy needed to sustain and maintain metabolism; it is the energy cost of living. The BMR describes “the rate of energy expenditure that occurs in the postabsorptive state, defined as the particular condition that prevails after an overnight fast, that subject having not consumed food for 12–14 hours and resting comfortably, supine, awake, and motionless in a thermoneutral environment. This standardized metabolic state corresponds to the situation in which food and physical activity have minimal influence on metabolism.”11 Basal energy expenditure (BEE), expressed as kcal/24 h, is the BMR extrapolated to 24 hours.
Total energy expenditure (TEE) in healthy subjects is made up of three components: REE (and thus BEE), AEE, and DEE.11
Resting energy expenditure (REE), or energy expenditure under resting conditions; REE is about 60% of TEE. Note that REE is made up of three variables: Basal energy expenditure (BEE), thermoregulation, and energy expended in depositing new tissue. As such, REE is usually about 10–20% higher than BEE. REE therefore includes all the homeostatic reactions in the human body.
Activity induced energy expenditure (AEE), which is about 30% of TEE. AEE is highly variable, depending on baseline physical activity level as well as a person’s physical capacity. For example, in obese patients, with a decreased level of activity, an AEE estimate of 30% TEE is accepted to be too high. However, in very active individuals, AEE can be over 50% of TEE.11
Diet-induced energy expenditure (DEE), which is about 10% of TEE. This is also referred to as the thermic effect of food (TEF).
From these three components, we see that four factors determine an individual’s daily energy requirements: gender, age, body size, and level of activity. Males require more kilocalories than females; the young require more kilocalories than the old; large people with more body mass require more kilocalories than smaller individuals; and the more active you are the higher your kilocaloric needs.
For example, a nonactive, frail 85-year-old lady who weighs less than 100 lb and is 4 ft tall requires only 1000 kcal/d, while a college-age male athlete weighing 220 lb and standing over 6 ft tall needs over 3000 kcal/d. On average, most adult men need about 2500 kcal/d and most adult women need about 2000 kcal/d.
Estimated energy requirements (EER) should, as far as possible, be based on estimates of energy expenditure. Energy expenditure can be derived in two ways: direct measurement or predictive equations (mathematical formulas).
The first method to determine EER is by direct measurement of TEE. This can be done by whole body calorimetry (the gold standard), indirect calorimetry, or the doubly labeled water (DLW) technique. These methods are rarely used nowadays and are not discussed in this chapter; a thorough discussion can be found elsewhere.11,12
The second method to determine EER is by predictive equations that estimate TEE. Three common formulas are: a simplified approach using ideal body weight, a more complex and traditional approach using the Harris-Benedict equation (rarely used clinically anymore but presented for its historical significance), and a more modern predictive equation developed by the Institute of Medicine (IOM). Other excellent predictive equations not presented here include the Mifflin-St Jeor equation13 and the Penn State equations.14,15
Ideal body weight TEE equation
The easiest formula to use to calculate energy requirements is based on a predictive equation for TEE using a patient’s ideal body weight (IBW):
This will give you a person’s kilocalorie energy needs over 24 hours. To find a person’s IBW, IBW tables exist as do user-friendly smartphone applications. This simple formula is useful for clinical purposes as an initial estimate. Given the components of TEE, however, the fixed value of 30 kcal/kg will vary depending on the clinical circumstances, such as during the post-injury stress response or after a major burn.
It is important to know that IBW, much like daily caloric needs, is derived from a calculation. There are various methods to calculating IBW; a few of the more well-known methods to calculate IBW were derived by Lorentz in 1929,16 Robinson in 1983,17 Devine in 1974,18 and Hamwi in 1964.19 They all produce approximately the same value of IBW,20 and are all derived by calculations based on height and gender. IBW should not be confused with two other types of body weight calculations, namely the adjusted body weight (ABW)21 and the lean body weight (LBW).22
While IBW is recommended in this simplified predictive formula for calculating kilocaloric needs, there are exceptions. In obese patients, IBW is much lower than their actual body weight. If a patient is overweight to the point that actual body weight is greater than 30% over calculated IBW, the adjusted body weight (ABW) is recommended for determining daily caloric needs (ABW can also be found in tables, smartphone/tablet applications, or online calculators).
LBW, meaning the total weight of all tissue in the body excluding fat (sometimes also referred to as fat-free mass, FFM), is the weight measure most closely correlated to BEE, REE, and TEE.11 LBW explains about 70–80% of the variance in energy expenditure. The other 20–30% is affected by age, gender, baseline nutritional state, inherited variations, and different endocrine states.
LBW is extremely variable at different stages of life. For example, from birth to adulthood: the brain increases its LBW fivefold; the liver, heart, and kidneys, which are even more metabolically active, increase 10- to 12-fold; muscle multiplies its LBW by about 40-fold.23 The lean tissue content of the body declines with age, and this accounts in part for a progressive fall in basal metabolic rate in relation to body size.24 In the elderly there is a decrease in the proportion of skeletal mass, as well as muscle.25 For all of these reasons, IBW is used to predict energy expenditure, not LBW.
There are times when it is important to calculate a healthy patient’s basal energy requirements (BEE). To do this via the IBW method, a patient’s BEE would be
BEE = IBW (in kg) × 24 kcal/kg
BEE is thus IBW multiplied by a smaller factor of 23–24 kcal/kg. TEE equation of 30 kcal/kg represents a 25% increase over BEE of 24 kcal/kg.26,27 Very limited activity increases the requirement to 28 kcal/kg.
Harris-Benedict equation for BEE
The Harris-Benedict equation (HBE)28 is perhaps the best known equation to determine a patient’s total kilocaloric needs over a 24-hour period. The equations were derived using indirect calorimetry. There are separate equations for males and females, and each is based on weight (in kilograms), height (in centimeters), and age (in years). The HBE equations predict BEE (not TEE):
Once the BEE has been calculated from the HBE, one needs to apply an activity factor multiplier to determine the TEE; the predicted value for TEE is then used as the EER for 24-hour kilocalorie needs. To do this, the baseline HBE values are multiplied as follows; the multipliers are the same for males and females29:
Institute of Medicine TEE equations
The Institute of Medicine (IOM) has published a set formula that predicts TEE based on age, height, weight, and physical activity level (PAL). They were derived using the DLW method for directly measuring TEE, and represent a more modern approach to determining estimated energy requirements (EER)11 (the IOM equations were defined in the year 2005 while the HBE was derived nearly 100 years ago, in the year 1918).28 These IOM TEE equations have replaced the Schofield Equation30 to define and develop the FDA’s dietary guidelines and formulate RDAs.
The IOM TEE prediction formula to calculate EER for males ages 19 years and older is (with age in years, weight in kilograms, and height in meters):
where PA is the physical activity coefficient:
PA = 1.00 if sedentary
PA = 1.11 if low active
PA = 1.25 if active
PA = 1.48 if very active
The IOM TEE prediction formula to calculate EER for females ages 19 years and older is (with age in years, weight in kilograms, and height in meters):
where PA is the physical activity coefficient:
PA = 1.00 if sedentary
PA = 1.12 if low active
PA = 1.27 if active
PA = 1.45 if very active
It has been suggested that the HBE and IOM equations for TEE do not add that much more accuracy beyond the simple IBW equations; this is confirmed in multiple guidelines which base TEE recommendations on predictive formulas.31,34 Please see Table 60-1 to get a sense for how these equations produce different EER estimates.
Methoda | Femaleb | Malec |
---|---|---|
IBW-based TEE | 1695 | 2100 |
HBE (baseline BEE) | 1429 | 1799 |
HBE TEE, sedentary | 1715 | 2159 |
HBE TEE, low activity | 1965 | 2474 |
HBE TEE, moderate activity | 2216 | 2789 |
HBE TEE, very active | 2466 | 3104 |
IOM TEE, sedentary | 1907 | 2529 |
IOM TEE, low activity | 1984 | 2672 |
IOM TEE, moderate activity | 2081 | 2854 |
IOM TEE, very active | 2197 | 3152 |
Protein
Proteins are large, nitrogenous organic compounds that are made up of long chains of amino acids; there are 20 different amino acids in our body’s proteins. Proteins exist in every cell, tissue, and organ in the human body, and are an essential part of all living organisms. Proteins are major structural components of muscle, bones, skin, and collagen; along with amino acids, proteins function as enzymes, antibodies, membrane receptors, hormones, and carriers of nutrients in the blood. Protein balance is often referred to as nitrogen balance.
Humans obtain proteins solely from the diet; once ingested, proteins are digested into their component amino acids. Dietary proteins come in two general forms: complete proteins and incomplete proteins. Complete proteins (aka whole protein; high-quality protein) come from animal-based products and supply all the amino acids, most importantly a group called the essential amino acids. There are nine essential amino acids (aka indispensable amino acids) which cannot be synthesized de novo by humans: histidine, isoleucine, leucine, lysine, methionine, phenylalanine, threonine, tryptophan, and valine. Incomplete proteins come from plants and lack one or more of the nine essential amino acids. When two or more incomplete proteins are combined to provide adequate amounts of all nine essential amino acids, they are referred to as complementary proteins.
Unlike fat (stored as adipose tissue) and carbohydrates (stored as glycogen in the liver and skeletal muscle), protein is not stored in the body. In contrast to other sources of energy, if more protein is ingested than is needed for metabolic purposes, all that excess nitrogen is metabolized and the end products are excreted. If less protein is ingested than is needed for homeostasis, leading to a persistent negative nitrogen balance, the body adapts by breaking down muscle, leading to a loss of LBW.10 See Box 60-2.
What is a safe level of intake of dietary protein per 24 hours, and how are the nutritional protein needs determined? The protein requirement of an individual is defined as the lowest level of dietary protein intake that will balance the losses of nitrogen from the body (principally in the urine, but also in feces and through the skin) in persons maintaining energy balance at modest levels of physical activity.23 Furthermore, we cannot simply maintain nitrogen balance; rather we must ensure that the required dietary protein intake includes both the essential amino acids and the nonessential amino acids. The amount of essential amino acids required in a healthy adult diet is 27.7%.35
There are two general methods for determining the daily recommended allowance for protein: direct measurement or predictive mathematical formula. The first method is to measure nitrogen loss, when the diet contains no protein, which will provide an estimate of nitrogen requirement. This has been done in nitrogen balance studies by collection of nitrogen losses in the urine, feces, skin, and sweat/secretions and subtracting these losses from measured nitrogen content of protein intake; the process is repeated multiple times, across several levels of protein intake, over many days, each time ensuring metabolic steady state has been reached. This process is quite impractical, though it is this process which forms the basis for estimating protein requirements using the predictive formula methodologies.10
The second method is by one of two different mathematical formulas: the first based on a person’s weight, the second based on daily energy requirements.
Protein requirement using weight-based method
Determining dietary protein needs for healthy subjects using IBW is based on long-term nitrogen balance studies.35 Specific equations have been derived from these studies, by both the World Health Organization36 as well as the Institute of Medicine,37 which provide nearly identical results. The Recommended Dietary Allowance (RDA) equation is 36,37,38:
There are three factors that went into determining this 24 hour requirement value. The first is the average amount of high-quality protein needed to maintain nitrogen balance: 0.6 g protein/kg IBW/d. The second is a safety factor to ascertain that 95% of the healthy population’s protein needs are covered: 0.15 g protein/kg IBW/d. The third is a buffer to allow for intake of proteins which are not high-quality proteins: 0.05 g protein/kg IBW/d.35
The equation is the same for both healthy adult men and women. While differences in lean body weight (LBW) exist between genders, as well as older and younger, these differences are offset partially by differences in weight.37 Accordingly, there is simply one equation for all healthy adults.
Protein requirement based on energy need
The most recent dietary reference intakes (DRIs)11,37 for macronutrients are designed to reflect a broadened view of protein needs, in relation to carbohydrate and fat requirements. This method, termed “acceptable macronutrient distribution range” (AMDR), more clearly reflects the interrelation between the macronutrients and allows for some level of flexibility in diet planning. If an individual consumes below or above the AMDR range, there is potential to affect long-term health by increasing the risk of chronic diseases, as well as increasing the risk of insufficient intakes of essential nutrients.39 See Table 60-2 for AMDRs for all macronutrients.
The AMDR for dietary protein is a safe range of intake, from 10 to 35% of the total daily kilocalorie requirement.37 This affords the ability to provide protein intake in excess of the RDA but within the AMDR; this has spawned the term “flexible calories,” because a clinician can use the AMDR for all three macronutrients to create varied dietary plans while still achieving kilocalorie needs.38
This alternative to the weight-based approach to determining protein needs is therefore based on the calculation for daily energy requirements. For example, the AMDR for protein is 10–35%; the FDA bases the daily recommended value (DRV) for protein on all food labels in the United States on a 10% rule (the low end of the AMDR for protein): 10% of 24-hour energy needs (as measured in kilocalories) should come from protein.40 The equation for the 10% rule is
A simpler way to calculate this is
While the FDA uses 10%, which is at the RDA level of 0.8 g protein/kg/d, one needs to consider the patient circumstances, and thus consider other values within the AMDR for protein. For example, there is good data to show that physically active people should have protein intakes in the range of 1.2–2.0 g protein/kg/d (15–25% of energy from protein).41,42,43 There is other research which now demonstrates the benefits of increased protein intake in elderly adults to levels nearly double the RDA of 0.8 g protein/kg/d, the goal being to preserve lean body mass and promote functional ability with age.44,45,46
Macronutrient | AMDR (as a percent of 24-hour energy requirements)a |
---|---|
Carbohydrate | 45–65% |
Protein | 10–35% |
Fat | 20–35% |
n-3 polyunsaturated fatty acids (linolenic acid) | 0.6–1.2% |
n-6 polyunsaturated fatty acids (linoleic acid) | 5–10% |
BOX 60-2: The Relationship Between Energy and Protein
Conceptually, there can be confusion about the link between energy and protein. On the one hand, food energy includes the energy from protein, which makes sense because protein not only provides a source of nitrogen and amino acids for the body, it also provides energy in the form of 4 kcal/g protein. In fact, the FDA’s DRV for protein is based on 10% of kilocaloric energy coming from protein.16 However, on the other hand nutritionists separate energy requirements from protein requirements. This practice seems to imply that the energy derived from protein is not included in the total energy needs—which is not true.
In practice, the total energy requirement (as determined by TEE) is calculated first, with no differentiation in how that energy will be derived (all three of the macronutrients can supply energy in the form of kilocalories). Next, the requirement for protein is calculated; one method to determine daily protein requirements is as a percentage of the TEE (see “Protein” section for specifics). In this manner, total energy requirements are determined independently of protein requirements, though protein requirements can be dependent on the total energy requirement calculation.
Energy and protein are fundamentally different in terms of how the body maintains and stores each. For energy, an individual’s intake must match his output if he is to remain in a steady state, and physiological mechanisms exist by which this balance is normally maintained. For example, when energy intake is below the requirement level, the body uses stores of energy to adapt. The opposite is true as well: when energy intake is above the required level, the body will store that excess energy, mostly as fat.
For protein, in contrast, there is no regulatory mechanism that matches intake to requirement. This is partly due to the fact that humans do not have protein reserves in the same way we have energy stored as glucose and fat; we are therefore dependent on protein intake to achieve required levels, as there are no protein stores to break down. And if we take in more protein than meets the needs of the body, then this excess nitrogen is excreted.
The body therefore handles excess protein very differently compared to how it handles excess energy. This is a fundamental concept, and these considerations have led to very different approaches to deciding the amount of dietary protein to maintain nitrogen balance versus deciding the amount of dietary energy to maintain energy balance.
For energy requirements, one intake achieves energy balance; we therefore determine the average requirement for energy.
For protein requirements, on the other hand, there is a range of intakes to choose from to achieve nitrogen balance; we therefore determine one intake within a safe range of intake for protein.11
Carbohydrates
Carbohydrates are organic compounds which contain only carbon, hydrogen, and oxygen atoms in a ratio usually of 1:2:1; most carbohydrates, but not all, follow the general chemical formula is Cn(H2O)n. What distinguishes one carbohydrate from another is the different ways the atoms combine.
Carbohydrates (aka carbs) are the most important energy source for humans, particularly the brain, which is a carbohydrate-dependent organ. In this manner, carbs can be thought of as the storage and transportation form of energy. Carbohydrates, however, are more than simply energy. Carbs also play major roles in our immune system, reproductive system, blood clotting, and cell structure; DNA and RNA are carbohydrate-based chains of molecules. The chemistry behind the structure of sugars is extremely complicated, and some have maintained that the genome is simple compared to the “glycome.” The study of the glycome is referred to as “glycomics,” and the science of carbohydrates’ role in life is termed “glycobiology.”
Carbohydrates are often referred to as sugars, or saccharides. Naturally occurring saccharides are produced by photosynthetic plants. Unnatural sugars (aka processed sugars; refined sugars; artificial sweeteners) are simply chemical compounds made in a lab, and lack vitamins and minerals; they are referred to an “empty calories.” The body digests most carbohydrates in the diet into glucose (C6H12O6; aka blood sugar). Glucose is then used in two ways: either as a source of energy via cellular respiration or stored as glycogen in the liver, skeletal muscle, and other tissues (excess glucose can also be stored as fat).
A monosaccharide is a carbohydrate in its elemental form, with one sugar unit. When two monosaccharides, or sugar units, combine they form a disaccharide. When three to ten monosaccharides join they form an oligosaccharides, which have complex, varied, and critical biological functions. Polysaccharides are built from any combination of monosaccharides and disaccharides; examples of polysaccharides are glycogen, the storage form of glucose, and starch.
Carbs come in two types: simple or complex, the difference being their underlying chemical structure. Simple carbohydrates are sugars in their simplest form, either one (monosaccharides) or two (disaccharides) sugars. Examples of monosaccharides include glucose, fructose (aka fruit sugar), and galactose; examples of disaccharides include sucrose (aka table sugar) and lactose (aka milk sugar).
Complex carbohydrates are made up of three or more simple carbohydrates bonded together into one larger compound; polysaccharides and oligosaccharides are complex carbs. Complex carbs come in two dietary forms: starch and dietary fiber. Starches are digested into sugars for energy; in addition to providing kilocalories, starches also contain vitamins and minerals. Complex carb-containing foods are popularly referred to as “starchy” foods.
Dietary fibers (aka roughage; bulk; nondigestible carbohydrates) are plant-derived complex carbs that the human body cannot digest or absorb, meaning fiber traverses the GI tract without being broken down. The health benefits of fibers are numerous, and include helping to control weight by causing satiety, aiding digestion, preventing constipation, attenuating blood glucose levels, and decreasing serum cholesterol.37 There are two general types of fiber: soluble and insoluble. Soluble fiber dissolves in water, and forms a gel-like substance in the GI tract that helps to lower blood cholesterol and glucose levels. Insoluble fiber doesn’t dissolve in water, and therefore promotes movement of material through the GI tract and helps with constipation and laxation.
What is an average requirement for carbohydrates per 24 hours, and how are the nutritional needs for carbohydrates determined? In general, carbohydrate needs are determined as a percentage of 24-hour energy requirements.11,37
Carbohydrate requirement based on energy needs:
The RDA for carbohydrates is based on a minimum amount necessary to cover the glucose needs of the carbohydrate-dependent central nervous system.11 With this in mind, when the FDA set the DRV for carbohydrates on all food labels in the United States, they used a very conservative value of 60%: 60% of 24-hour kilocalorie energy needs should come from carbohydrates.40
A more modern approach to determining daily carbohydrate needs is to consider the Institute of Medicine’s Acceptable Macronutrient Distribution Range (AMDR) for carbohydrates.11 The AMDRs delineate upper and lower bounds for the percentage of daily calories provided from all macronutrients; these safe ranges recognize the interrelation between the macronutrients, and allow flexibility in creating customized diets to individual patient needs.
For carbohydrates, the AMDR is from 45 to 65% of total daily kilocalorie requirement (see Table 60-2).37 This means that carbohydrate-derived energy can safely make up anywhere from 45 to 65% of total energy needs. The calculation for determining 24-hour carbohydrate requirements is based on 4 kcal of energy being produced from every gram of carbohydrate consumed (note that for parenteral nutrition calculations the conversion is 3.4 kcal/g). For example, using the FDA’s DRV of 60%, the equation is
To determine other values, one simply substitutes for any percentage, from 45 (use 0.45 in the equation) to 65% (use 0.65 in the equation).
Fat
Fats are an essential nutrient, and a key part of our diet. They are characterized by being insoluble in water, nonvolatile, and greasy to touch. Fats are sometimes referred to as lipids, though technically speaking fats are a member of the lipid family.
Lipids are a group of naturally occurring molecules that are derived from one of two common biochemical subunits (ketoacyl or isoprene). Lipid compounds include fatty acids (from which fats are derived); glycerolipids (triglycerides; diglycerides; monoglycerides; collectively aka glycerides); glycerophospholipids (aka phospholipids); sterol lipids (such as cholesterol and steroids); saccharolipids (such as lipopolysaccharides in gram negative bacteria); and others (see Ref. 11 for more details).
Fats provide a needed source of energy for the human body. As an energy source, fat produces 9 kcal energy per gram, which is over twice as many kcal as can be derived from carbohydrates (4 kcal/g enterally; 3.4 kcal/g parenterally) and protein (4 kcal/g). For this reason fat is referred to nature’s storehouse of energy. Fats are necessary for the body to absorb the fat-soluble vitamins A, D, E, and K. Fats help insulate the body, and keep skin and hair healthy.
Fats also provide humans with the essential fatty acids: linoleic acid and linolenic acid (note that linoleic acid is the precursor to arachidonic acid, so technically speaking arachidonic acid is not considered essential). Humans can synthesize all but these two essential fatty acids. Fats can be synthesized from the breakdown products of proteins, carbohydrates, or other fats (using acetate as an intermediate metabolite).
The essential fatty acids are crucial for membrane structure lipids, cell-signaling pathways, brain development, controlling inflammation, and blood clotting. Prostaglandins are hormone-like compounds derived from arachidonic acid, and are involved in many vital functions in the human body.
As a chemical compound, fats contain carbon, hydrogen, and oxygen with the defining feature that they are arranged as a hydrocarbon chain skeleton, with a carboxyl group (-COOH) at one end and a methyl group (CH3-) at the other. Fats are made from various fatty acids, which contribute approximately 95% of the total weight to various fats; the other 5% is from a backbone molecule to which the fatty acids bond, glycerol (and other occasionally attached molecules). Fatty acids are differentiated by the number of carbon atoms in their carbon skeleton as well as the number of carbon to carbon (C=C) double bonds. These differences lead to variations in structure and function. There are more than 100 different fatty acids in the human body, though less than 20 contribute to the majority of fats.
Fats are classified as either unsaturated or saturated. Unsaturated fats have at least one C=C double bond in the carbon skeleton, which gives them a low melting temperature. They are often liquids at room temperature, known as oils. There are two types of unsaturated fats: monounsaturated and polyunsaturated. Monounsaturated fats have only one C=C double bond in the carbon skeleton. Polyunsaturated fats have two or more C=C double bonds in the carbon skeleton. Polyunsaturated fats include the two essential fatty acids: omega-3 polyunsaturated fats (linolenic fatty acid) and omega-6 polyunsaturated fats (linoleic fatty acid).
Synthetic fats are unnatural, unsaturated fats created in an industrial lab when hydrogen is added to liquid vegetable oil (a natural unsaturated fat) to create solid fat. These fats are used mainly as preservatives for food and to add texture. Synthetic fats come in two types: hydrogenated fats and partially hydrogenated fats (aka trans fats; trans fatty acids). Trans fats have been proven to increase cholesterol and the risk for heart disease,47,48 and have a detrimental effect on the brain and nervous system49; in short, they have no benefit to human health.11 It should be noted that there are some trans fats that are naturally occurring, found in animal fats.
Saturated fats are so named because the carbon skeleton in these fats is saturated with hydrogen bonds, and therefore have no C=C double bonds. These fats are solids at room temperature, indicating a high melting temperature. They are generally referred to as the solid fats and come from two general sources: the primary source is animals, including meats and dairy products (all animal-based saturated fats also contain cholesterol). The second source is certain plants. Saturated fats were once thought to be linked to coronary heart disease and increased cholesterol levels, though that is being questioned by more recent data.50
Cholesterol is an organic sterol lipid molecule, synthesized by all animal cells. Cholesterol is an essential component of animal cell membranes, and is the precursor to steroid hormones, bile salt (it was first discovered in gallstones), and vitamin D. It is found in all foods containing animal fat; cholesterol is not found in significant amounts in plant sources.
Triglycerides are lipid compounds derived when one molecule of glycerol combines with three fatty acids. Triglycerides are the major storage form of fat in human adipose tissue; the hydrolysis of triglyceride ester bonds is the first step in fat metabolism.
What is an average requirement for fat per 24 hours, and how are nutritional fat needs determined? Neither an adequate intake (AI) level nor a Recommended Daily Allowance (RDA) exists for total fat. This is because there is not sufficient data to determine a level below which intake is inadequate or above which chronic diseases are prevented.11 The acceptable macronutrient distribution range (AMDR) for fat for healthy adults is 20–35% of the total daily kilocalorie requirement.37 Accordingly fat needs are determined as a percentage of 24-hour energy requirements.
Fat requirement based on energy needs
The AMDR for fat is 20–35% (see Table 60-2). This range was set to ensure that the essential fatty acids were consumed in adequate amounts. The FDA has based its DRV for fat on a value of 30%: 30% of the 24-hour energy needs (as measured in kilocalories) should come from fat.40 The equation to calculate 24-hour fat requirements is (using 30%, which can change depending on the percentage desired):
To ensure intake of adequate dietary amounts of the essential fatty acids, approximately 10% of total daily kilocalorie intake should come from longer-chain polyunsaturated fats (see Table 60-2)37:
Omega-3 polyunsaturated fat (linolenic fatty acid) intake should be in the range of 0.6–1.2% of 24-hour kilocalorie needs
Omega-6 polyunsaturated fats (linoleic fatty acid) should be in the range of 5–10% of 24-hour kilocalorie needs
Current, dietary recommendations also maintain that humans should consume less than 10% of total energy from saturated fats.
Water
The human body is made up of 60% water, and on average, 60% of an adult’s total body weight is water.51 Water is the basis of all fluids in our body: for example, bile is 90% water; blood is 92% water; cerebrospinal fluid is 99% water. Water is also the basis of all organs and tissues in our body: bone is 31% water; the brain and heart are 73% water; muscles and kidneys are 79% water; and lungs are 83% water.52 Total body water (TBW) is distributed between the intracellular fluid (ICF; 65% TBW) and extracellular fluid (ECF; 35% TBW); of the TBW in the ECF, 79% is in the interstitial space and 21% is intravascular in plasma.
Water is made up of three atoms: two hydrogen and one oxygen. The simplicity of the water compound belies its importance, its versatility, and its complexity, both in terms of its physical and chemical properties. Water is the solvent, the medium, and the participant in most biochemical and physiological reactions occurring in our bodies; it absorbs and releases metabolic heat; it is attracted to itself and many other substances, and has tremendous surface tension and strength.
Human life and cellular homeostasis depend on water: we could live roughly a month without macronutrients; we can live only a few days without water. Once reaching 10–14% dehydration, risk of death increases rapidly, approaching irreversibility.51 It is via water that carbohydrates and proteins are metabolized; aqueous solutions are the universal transporter of macronutrients, oxygen, and waste; cellular hydration is a crucial signal regulating cell metabolism and gene expression; water aids in digestion, is a lubricant for joints, and a shock absorber for the brain and spinal cord; water helps regulate body temperature through sweating and respiration.51,52
What is an average requirement for water per 24 hours, and how are the nutritional water needs determined? There is no RDA for water as there is not sufficient data to determine a level above which chronic diseases are prevented.51 The major risk associated with low intake of total water is dehydration, primarily in the acute setting, which can lead to metabolic and functional abnormalities. Accordingly, an Adequate Intake (AI) has been established for water below which intake is inadequate. These AIs were defined to cover minimal losses from temperate climates for a sedentary individual.
The AI for total water per 24 hours to prevent dehydration in adult men is 3.7 L and in adult women is 2.7 L51; 80% of this is typically consumed by liquids, meaning healthy adult men should drink 3.0 L (13 cups) per day and women 2.2 L (9 cups); the remaining balance of water comes from food. This intake of water will replace respiratory, urinary, fecal, and insensible fluid losses. Higher intakes should be achieved in physically active people or those exposed to hot environments.
Daily consumption of water below the AI may not translate into an added risk of dehydration because normal hydration can be achieved and maintained over a wide range of intake. Very importantly, the AI is therefore not a specific requirement.51 In healthy adults, fluid intake, driven by consumption of liquids and food at meals as well as by thirst, maintains total body water at normal levels, preventing dehydration. Body water balance is achieved when water gain and water loss are equal. Water gain occurs from consumption (sources of water include drinking water, water in beverages, and water that is part of food) and production (metabolic water). Water loss occurs from respiratory loss, urinary/renal loss, fecal/GI tract loss, skin loss, and insensible loss.
The interrelationship between the macronutrients, other substances we consume, and water demonstrates the interconnectedness of homeostatic processes in humans. Increased or decreased intake of macronutrient as well as these other substances (such as caffeine, alcohol, and sodium) can and will affect water requirements.39
For example, for dietary proteins and amino acids, the major end product of their metabolism is urea. Urea requires water for excretion by the kidneys. Therefore increased protein intake requires increased water intake. For dietary carbohydrates, 100 g/d are required to prevent ketosis; consuming fewer carbs can increase ketone bodies, which require water to be excreted. Therefore increased ketosis requires increased water. And lastly, fecal water losses are increased with higher loads of dietary fiber; with increased fiber intake more water needs to be consumed to prevent dehydration.
Micronutrients: vitamins and minerals
The term “micronutrients” is used to refer to both vitamins and minerals. Vitamins are organic substances made by plants or animals; they are essential for growth and development. Vitamin deficiencies can be seen in the elderly (especially frail or institutionalized people with malnutrition), alcoholics (with associated malnutrition), illicit drug users, impoverished populations, and those in developing countries.
There is a long list of vitamins that humans need; vitamins can be grouped together as follows:
Fat-soluble vitamins
vitamin A (retinol; including the carotenoids)
vitamin D
vitamin E
vitamin K
Water-soluble vitamins
B vitamins
biotin
folate (folic acid)
niacin (nicotinic acid)
pantothenic acid
riboflavin (vitamin B2)
thiamin (vitamin B1)
vitamin B6 (pyridoxine)
vitamin B12 (cobalamins)
vitamin C (ascorbic acid)
choline
Please see Table 60-3 for vitamin functions, deficiencies, and toxicities; please see Table 60-4 for dietary reference intakes for the vitamins.39,53
Minerals, on the other hand, are inorganic elements that come from the earth, soil, and water; all minerals are found in the periodic table of elements.54 Minerals are absorbed by pants from the ground, and humans consume minerals from eating these plants. Minerals are essential for human homeostasis, as the body uses them for normal bone, muscle, heart, and brain function as well as for making hormones.39
There is a long list of minerals that humans need. The minerals can be grouped together as follows:
Six macrominerals, which are needed in large amounts (in the range of grams per day); these are usually simply referred to as electrolytes (will be discussed in a later section):
Four cation electrolyte macrominerals:
Sodium (Na; element #11; an alkali metal)
Potassium (K; element #19; an alkaline earth metal)
Calcium (Ca; element #20; an alkaline earth metal)
Magnesium (Mg; element #12; an alkaline earth metal)
Two anion electrolyte macrominerals:
Chloride (Cl, chlorine; element #17; a nonmetal halogen gas)
Phosphorus (P; element #15; a nonmetal solid)
Nine trace minerals, which are needed in small amounts (in the milligram or less per day range):
Chromium (Cr; element #24; a transition metal)
Copper (Cu; element #29; a transition metal)
Fluoride (F, fluorine; element #9; a nonmetal halogen gas)
Iodine (I; element #53; a nonmetal halogen)
Iron (Fe; element #26; a transition metal)
Manganese (Mn; element #25; a transition metal)
Molybdenum (Mo; element #42; a transition metal)
Selenium (Se; element #34; a nonmetal solid)
Zinc (Zn; element #30; a transition metal)
Other minerals used by the body (not discussed in depth in this chapter; for more information see IOM report referenced here39)
Sulfate (S, sulfur; element #16; a nonmetal solid)
Arsenic (As; element #33; a metalloid)
Boron (B; element #5; a metalloid)
Nickel (Ni; element #28; a transition metal)
Silicon (Si; element #14; a metalloid)
Vanadium (V; element #23; a transition metal)
Please see Table 60-5 for trace mineral functions, deficiencies, and toxicities39,55; please see Table 60-6 for DRIs for the trace minerals.39,55
Nutrient | Vitamin functions | Effect of deficiency | High risk deficiency patients & notes | Effect of toxicity |
---|---|---|---|---|
Fat-soluble vitamins | ||||
Vitamin A (retinol) | Important for vision (formation of rhodopsin, a photoreceoptor in the retina), gene expression, reproduction, embryonic development, growth, immune function. Also integrity of epithelia; lysosome stability; glycoprotein synthesis | Xerophthalmia (an irreversible drying of the conjunctiva and cornea) leading to night blindness; perifollicular hyperkeratosis; keratomalacia; increased morbidity & mortality in children | People with high alcohol intake, preexisting liver disease, hyperlipidemia, or severe protein malnutrition may not be protected by the UL set for the general population, because the requirements for vitamin A are based on the assurance of adequate liver stores of vitamin A | Hypervitaminosis A may be acute or chronic: headache; peeling of skin; hepatosplenomegaly; bone thickening; intracranial hypertension; papilledema; hypercalcemia; teratogenicity |
Vitamin D | Involved in bone health (mineralization & repair). It aids in the absorption of calcium and phosphorus in the tubules of the kidneys, thereby helping maintain normal serum levels of these minerals. Also insulin and thyroid function, improvement in immune function, reduced risk of autoimmune disease | Impair normal bone metabolism, which may lead to rickets in children and osteomalacia in adults. It is also implicated in osteoporosis in adults | Older adults, especially those who live in northern industrialized cities of the world, are more prone to developing vitamin D deficiency (due to lack of sunlight) | Hypervitaminosis D: Hypercalcemia, hypercalciuria, and calcification of soft tissues, such as blood vessels and certain organs |
Vitamin E | Functions as a chain-breaking antioxidant in the body by preventing the spread of free-radical reactions. Is an intracellular antioxidant; scavenger of free radicals in biologic membranes | Peripheral neuropathy | Generally occurring only as the result of genetic abnormalities of vitamin E metabolism, fat malabsorption syndromes, or protein-energy malnutrition | Hemorrhagic toxicity (tendency to bleed) |
Vitamin K | Functions as a coenzyme for biological reactions involved in blood coagulation (formation of prothrombin, other coagulation factors) and bone metabolism | Classic sign of vitamin K deficiency is a vitamin K–responsive increase in prothrombin time and, in severe cases, bleeding; osteopenia | Clinically significant vitamin K deficiency is extremely rare in the general population, with cases being limited to individuals with malabsorption syndromes or those treated with drugs known to interfere with vitamin K metabolism | |
Water-soluble vitamins | ||||
B Vitamins | ||||
Biotin | Functions as a coenzyme in bicarbonate-dependent carboxylation reactions | Dermatitis, alopecia, conjunctivitis, and abnormalities of the central nervous system | Individuals consuming raw egg whites over long periods and in patients receiving total parenteral nutrition (TPN) solutions that do not contain biotin | NA |
Folate (folic acid) | Functions as a coenzyme in the metabolism of nucleic and amino acids (purines, pyrimidines, and methionine); maturation of red blood cells; development of fetal nervous system | Decreased erythrocyte folate concentratin leads to macrocytic anemia (first evidenced by a low erythrocyte count and eventually by a low hematocrit and hemoglobin); neural tube birth defects; confusion | Coexisting iron or vitamin B12 deficiency may interfere with the diagnosis of folate deficiency. To reduce the risk of neural tube defects, women able to become pregnant should supplement folic acid | NA |
Niacin (nicotinic acid) | Involved in many biological reactions (oxidation-reduction reactions), including intracellular respiration and fatty acid synthesis; carbohydrate and cell metablism | The classic disease of severe niacin deficiency is Pellagra (dermatitis, glossitis, GI and CNS dysfunction), which in industrialized nations generally only occurs in people with chronic alcoholism or conditions that inhibit the metabolism of tryptophan | People with an increased need for niacin include those with Hartnup’s disease, liver cirrhosis, carcinoid syndrome, and malabsorption syndrome, as well as those on longterm isoniazid treatment for tuberculosis or on hemodialysis or peritoneal dialysis. Also, pregnant females who are carrying more than one fetus or breastfeeding more than one infant may require additional niacin | Flushing, nausea and vomiting, liver toxicity, and impaired glucose tolerance. However, most of the data on adverse effects has come from research with patients with special conditions who were treated with pharmacological preparations |
Pantothenic acid | Functions as a component of coenzyme A (CoA), which is involved in fatty acid metabolism | Irritability & restlessness, fatigue, apathy, malaise, sleep disturbances, hypoglycemia; neurobiological symptoms, such as numbness, paresthesias, muscle cramps, and staggering gait | Pantothenic acid deficiency is rare and has only been observed in individuals who were fed diets devoid of the vitamin or who were given a pantothenic acid metabolic antagonist | NA |
Riboflavin (vitamin B2) | Functions as a coenzyme in numerous oxidation–reduction reactions in several metabolic pathways (especially carbohydrate and protein metabolism) and in energy production; integrity of mucous membranes | Sore throat, hyperemia and edema of the pharyngeal and oral mucous membranes, cheilosis, angular stomatitis, glossitis, seborrheic dermatitis, and normocytic anemia associated with pure erythrocyte cytoplasia of the bone marrow | Riboflavin deficiency is most often accompanied by other nutrient deficiencies, and it may lead to deficiencies of vitamin B6 and niacin, in particular. Diseases such as cancer, cardiac disease, and diabetes mellitus are known to precipitate or exacerbate riboflavin deficiency | NA |
Thiamin (vitamin B1; aneurin) | Functions as a coenzyme in the metabolism of carbohydrates, fat, branched-chain amino acid, glucose, and alcohol metabolism; central and peripheral nerve cell function; myocardial function | Beriberi (peripheral neuropathy, heart failure); Wernicke-Korsakoff syndrome | The classic disease of thiamin deficiency is beriberi, which is sometimes seen in developing countries. Severe thiamin deficiency in industrialized nations is often associated with chronic heavy alcohol consumption and presents as Wernicke-Korsakoff syndrome | NA |
Vitamin B6 (pyridoxine) | Function as a coenzyme in the metabolism of amino acids (protein, nitrogen), glycogen, and sphingoid bases (transaminations, porphyrin & heme synthesis, tryptophan conversion of niacin) | The signs and symptoms of vitamin B6 deficiency are seborrheic dermatitis, microcytic anemia, epileptiform convulsions, and depression and confusion | Sensory peripheral neuropathy & dermatological lesions | |
Vitamin B12 (cobalamins) | Functions as a coenzyme for a reaction that converts homocysteine to methionine & for a separate reaction in the metabolism of certain fatty acids and amino acids; maturation of red blood cells; neural function; DNA synthesis; myelin synthesis and repair | Macrocytic anemia; neurologic deficits (confusion, parethesias, ataxia) | The major cause of vitamin B12 deficiency is pernicious anemia, a condition in which the gastric mucosa of the stomach does not produce intrinsic factor. The hematological effects that occur with this deficiency are identical to those observed in folate deficiency | NA |
Vitamin C (ascorbic acid) | Acts as an antioxidant and a cofactor in enzymatic and hormonal processes. It also plays a role in the biosynthesis of carnitine, neurotransmitters, collagen, and other components of connective tissue (bone and blood vessel health & wound healing), and modulates the absorption, transport, and storage of iron | The classic disease of severe Vitamin C deficiency is Scurvy (follicular hyperkeratosis, petechiae, ecchymoses, coiled hairs, inflamed and bleeding gums, perifollicular hemorrhages, joint effusions, arthralgia, and impaired wound healing) | Severe vitamin C deficiency is rare in industrialized countries, but it is occasionally seen in people whose diets lack fruits and vegetables or in those who abuse alcohol or drugs | Diarrhea & other gastrointestinal disturbances |
Choline | Required for the structural integrity of cell membranes. It is also involved in methyl metabolism, cholinergic neurotransmission, transmembrane signaling, and lipid and cholesterol transport and metabolism | Liver damage | Few data exist on the effects of inadequate dietary intake in healthy people | Fishy body odor (trimethylaminuria), sweating, salivation, hypotension, and hepatotoxicity in humans |
Nutrient | Females (age range in years) | Males (age range in years) | ULb | ||||||||
---|---|---|---|---|---|---|---|---|---|---|---|
14–18 | 19–50 | 51–70 | 71+ | 14 –18 | 19–70 | 51–70 | 71+ | 14–18 | 19–70 | 71+ | |
Fat-soluble vitamins | |||||||||||
Vitamin A (retinol; mcg RAE/day)c | 700 | 700 | 700 | 700 | 900 | 900 | 900 | 900 | 3000 | 3000 | 3000 |
Vitamin D (mcg/day)d | 5 | 5 | 10 | 15 | 5 | 5 | 10 | 15 | 50 | 50 | 50 |
Vitamin E (mg/day)e | 15 | 15 | 15 | 15 | 15 | 15 | 15 | 15 | 800 | 1000 | 1000 |
Vitamin K (mcg/day)d | 75 | 90 | 90 | 90 | 75 | 120 | 120 | 120 | – | – | – |
Water-soluble vitamins | |||||||||||
B Vitamins | |||||||||||
Biotin (mcg/day)d | 25 | 30 | 30 | 30 | 25 | 30 | 30 | 30 | – | – | – |
Folate (mcg/day)e | 400 | 400 | 400 | 400 | 400 | 400 | 400 | 400 | 800 | 1000 | 1000 |
Niacin (mg/day)e | 14 | 14 | 14 | 14 | 16 | 16 | 16 | 16 | 30 | 35 | 35 |
Pantothenic acid (mg/day)d | 5 | 5 | 5 | 5 | 5 | 5 | 5 | 5 | – | – | – |
Riboflavin (vitamin B2; mg/day)e | 1.0 | 1.1 | 1.1 | 1.1 | 1.3 | 1.3 | 1.3 | 1.3 | – | – | – |
Thiamin (vitamin B1; mg/day)e | 1.0 | 1.1 | 1.1 | 1.1 | 1.2 | 1.2 | 1.2 | 1.2 | – | – | – |
Vitamin B6 (pyridoxine; mg/day)e | 1.2 | 1.3 | 1.5 | 1.5 | 1.3 | 1.3 | 1.7 | 1.7 | 80 | 100 | 100 |
Vitamin B12 (cobalamins; mcg/day)e | 2.4 | 2.4 | 2.4 | 2.4 | 2.4 | 2.4 | 2.4 | 2.4 | – | – | – |
Vitamin C (ascorbic acid; mg/day)d | 65 | 75 | 75 | 75 | 75 | 90 | 90 | 90 | 1800 | 2000 | 2000 |
Choline (mg/day)d | 400 | 425 | 425 | 425 | 550 | 550 | 550 | 550 | 3000 | 3500 | 3500 |
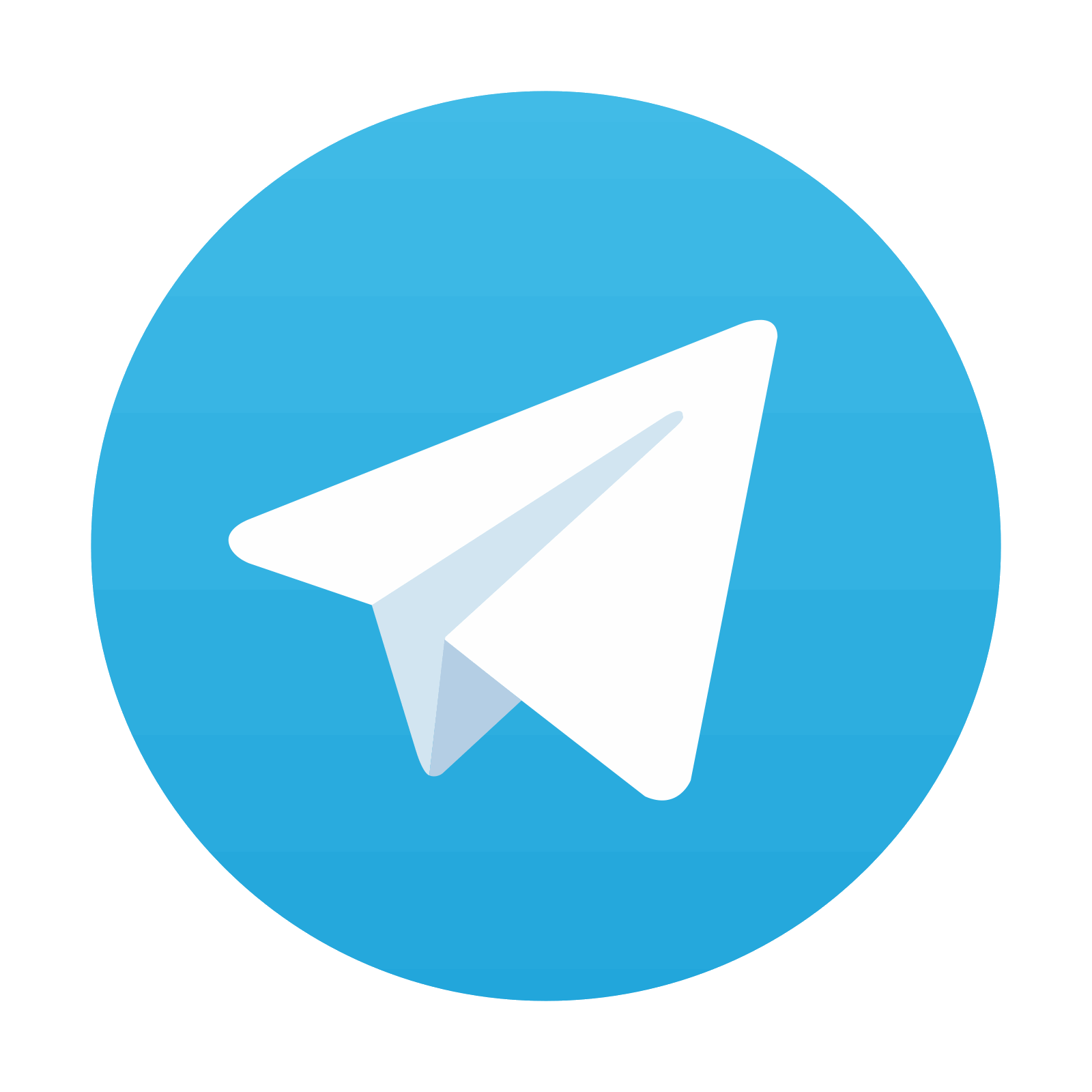
Stay updated, free articles. Join our Telegram channel
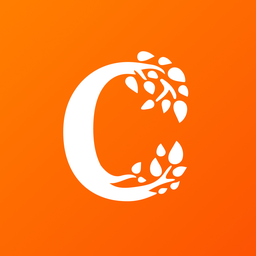
Full access? Get Clinical Tree
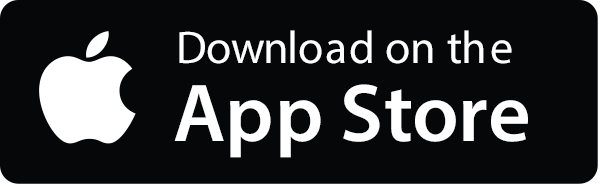
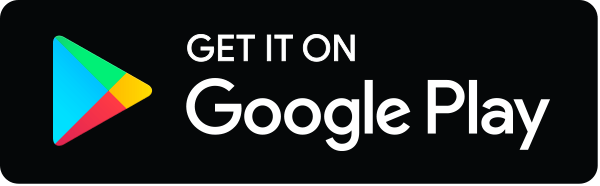
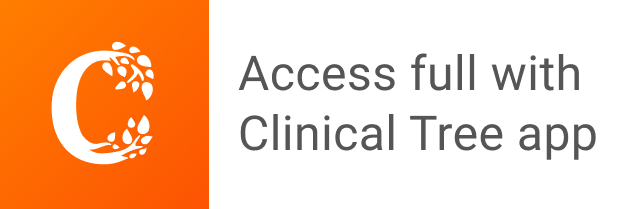