Fig. 15.1
7 T DCE. Invasive ductal carcinoma G2 in a 50-year-old woman at 1 o’clock in the right breast. (a) At DCE MRI of the breast at 7 T the irregular-shaped mass with spiculated margins (white arrow) demonstrates heterogeneous initial strong enhancement followed by a plateau (b), and was classified as BI-RADS© 5 (highly suggestive of malignancy)
15.2.3 Diffusion-Weighted Imaging
DWI is a non-contrast MRI parameter, which measures the random movement of water molecules, i.e., Brownian movement, and depicts the diffusivity of the examined tissues. DWI provides a strong surrogate marker for tissue microstructure, membrane integrity, and cell density, and can be quantified by calculating the apparent diffusion coefficient (ADC) [59]. Changes in tissue water diffusion properties can be helpful for the detection and characterization of pathological processes in any part of the body [59, 60]. New developments in imaging techniques (e.g., parallel imaging) and hardware (stronger gradient systems and multi-channel coils) have overcome previous limitations (susceptibility and respiratory motion artifacts) and DWI has been implemented in oncologic imaging in multiple organs. In general, malignant tissue tends to have more restricted diffusion and lower ADC values compared to normal tissue due to the high cellular density and abundance of intra- and intercellular membranes [61].
DWI for breast cancer diagnosis has been evaluated with encouraging results by numerous studies using different ADC thresholds and b-values [62–64]. Bogner et al. compared the diagnostic quality of DWI schemes with respect to ADC accuracy, ADC precision, and DWI contrast-to-noise ratio (CNR) for different types of lesions and breast tissue at 3 T [65]. They concluded that optimal ADC determination and DWI quality at 3 T was found with a combined b-value protocol of 50 and 850 s/mm2, yielding a diagnostic accuracy of 96 % (Fig. 15.2). In a recent meta-analysis including 26 studies, Dorrius et al. [66] confirmed that ADC values of breast lesions are strongly influenced by the choice of b values. For the most accurate differentiation of benign and malignant lesions, the combination of b = 0 and 1000 s/mm2 was recommended. Nevertheless, there is a consensus that, in general, breast cancer has lower ADC values compared to healthy breast tissue [43, 62, 67], that the specificity of DWI (75–84 %) is higher than DCE-MRI (67–72 %), and that DWI is a promising imaging biomarker that provides additional functional information to DCE-MRI [68]. DWI also can be easily integrated into a standard MR imaging protocol [11, 13, 69].
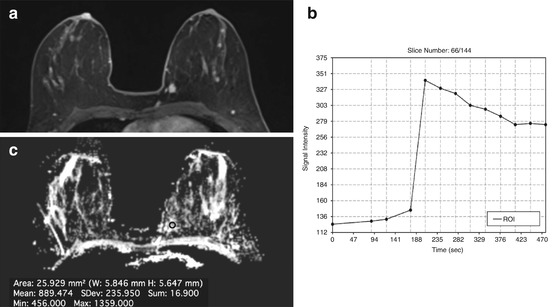
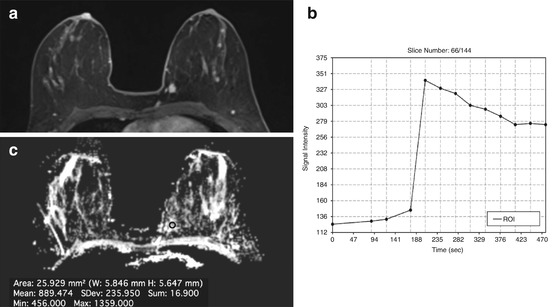
Fig. 15.2
Invasive ductal carcinoma (IDC) medially in the left breast in a 45-year-old woman. (a) The irregular shaped and marginated mass lesion demonstrates (b) an initial strong heterogeneous contrast enhancement followed by a wash-out, and had decreased (c) ADC values (0.889 × 10−3 mm2/s). DCE-MRI and DWI at 3 T were concordant and both classified the mass lesion as malignant
Several authors recommended cut-off thresholds to discriminate benign and malignant lesions [65, 69–73]. A very low ADC value (<1.25 × 10−3 mm2/s) is specific for malignancy, whereas high ADC values (>1.4 × 10−3 mm2/s) are indicative of benignity. Intermediate ADC values are rather unspecific. In a recent study, Spick et al. [74] applied hierarchized ADC thresholds to suspicious breast lesions only visible on DCE MR, and concluded that up to 35 % of MR-guided biopsies could have been omitted when using a high ADC threshold of >1.4 × 10−3 mm2/s.
In addition to the differentiation of benign and malignant breast tumors, DWI can potentially be used as a non-invasive biomarker for tumor receptor status and tumor grading. Martinicich et al. [75] found that breast cancers characterized by high cellularity or a higher number of mitoses have lower ADC values. Similar observations have been published about triple-negative breast cancers, which are associated with higher ADC values compared to ER+ and HER2/neu-enriched tumors [76–79]. In contrast, mucinous carcinoma usually presents ADC values similar to benign lesions, most likely due to the presence of both low cellularity and mucin-rich compartments [68]. Bickel et al. evaluated whether ADC with DWI at 3 T can be used as an imaging biomarker to differentiate invasive breast cancer from noninvasive ductal carcinoma in situ (DCIS). In that study, in addition to being used as an imaging biomarker for the diagnosis of breast cancer, ADC seemed to be a valuable noninvasive quantitative biomarker to assess breast cancer invasiveness, and thus, has the potential to reduce over-diagnosis and subsequent over-treatment [80]. Another promising application for DWI is the monitoring of breast cancer treatment. ADC values are very sensitive to changes in tumor cellularity and necrosis. The cytotoxic effect of anticancer drugs increases the Brownian movements in damaged tissues and is reflected by an increase in ADC values which occurs earlier than lesion size changes or vascularity, as measured with DCE-MRI, suggesting DWI can provide a valuable early indication of treatment efficacy [81, 82]. Park et al. [83] studied the potential of DWI in the prediction of response to neo-adjuvant chemotherapy in breast cancer patients. Patients with a low pretreatment ADC tended to respond better to chemotherapy. The best pretreatment ADC cutoff with which to differentiate between responders and nonresponders was 1.17 × 10−3 mm2/s, which yielded a sensitivity of 94 % and a specificity of 71 %. Richards et al. [84] found that pretreatment tumor ADC values differed between intrinsic subtypes and were predictive of pathologic response in triple-negative tumors. However, the literature presents divergent results for the assessment of pre-chemotherapy ADC, and more data to fully elucidate the role of ADC as a potential biomarker for predicting the response to neoadjuvant treatment in the breast is warranted [61, 82, 85, 86].
Several advanced modeling approaches for DWI to extract more biologically relevant information are currently under investigation.
Intravoxel incoherent motion (IVIM): DWI is also sensitive to perfusion because the flow of blood in randomly oriented capillaries mimics a diffusion process through the IVIM effect [87]. Several studies have investigated IVIM in breast tumors and preliminary data suggests that it can provide valuable information about both tissue microstructure and microvasculature that is beneficial for the diagnosis of breast cancer lesions [88–92].
Diffusion-Weighted Kurtosis (DKI): In living tissue, DWI is affected by Brownian incoherent motion and micro-perfusion or blood flow showing non Gaussian phenomena [93]. The diffusion-weighted kurtosis (DKI) quantifies the deviation of tissue diffusion from a Gaussian pattern and has demonstrated a substantially higher sensitivity and specificity in cancer detection compared with ADCs [94, 95].
Diffusion Tensor Imaging (DTI): DTI is considered to be an extension of DWI, which provides information about water motion in six or more directions, and thus, characterizes the motion of water in more detail [96, 97]. DTI provides measurements of two parameters: mean diffusivity (MD) and fractional anisotropy (FA). MD reflects the average anisotropy, whereas FA describes the degree of anisotropy [97, 98]. The diffusion of water molecules in the mammary glandular/ductal system presents an excellent example of restricted movement. There is free diffusion parallel to the walls of the ducts and lobules and a restricted diffusion in the perpendicular directions, leading to an anisotropic diffusion [99]. Based on histopathological data, most breast pathologies result in decreased structuring compared to healthy tissue. Therefore, any changes of this tissue structure by means of benign or malignant tumor growth should be reflected by changes in diffusion anisotropy detectable with DTI [96, 97, 99]. Partidge et al. [100] investigated whether DTI measures of anisotropy in breast tumors are different from those in normal breast tissue and whether this could improve the discrimination between benign and malignant lesions. The authors demonstrated that diffusion anisotropy is significantly lower in breast cancers than in normal tissue, which may reflect alterations in tissue organization, but that it cannot reliably differentiate between benign and malignant lesions. Baltzer et al. [96] proved that DTI can visualize micro-anatomical differences between benign and malignant breast tumors, as well as normal breast parenchyma. However, FA did not have an incremental value compared to ADC. Although results for the diagnostic accuracy of FA are divergent [96, 97, 101], it seems that DTI has the potential to serve not only as an adjunct method to DCE examination, but also as an alternative method when DCE imaging is contraindicated [96, 99, 100].
15.2.4 Proton MR Spectroscopy
MRS is a non-invasive technique that reflects the chemical composition of tissue by providing spatially localized signal spectra with spectral peaks representing the structure and concentration of different chemical compounds in the region of interest. Based on these signal spectra, which provide information about the varying levels of associated detectable metabolites, MRS is able to differentiate tissue conditions such as normal, benign, malignant, necrotic, or hypoxic. It has been demonstrated that, in breast imaging, the additional application of 1H-MRS aids in the characterization of breast tumors [102, 103]. In breast imaging, the additional value of 1H-MRS is based on the detection of choline (Cho). The Cho peak observed in vivo is located at approximately 3.2 ppm and is a composite of several different Cho-containing compounds, such as free choline, phosphocholine, and glycerophosphocholine, and is commonly referred to as total Cho (tCho). Cho is involved in cell membrane turnover and is thus considered an imaging biomarker of cell proliferation. In breast cancer, the elevated Cho signal is thought to result from a combination of increased intracellular phosphocholine concentration and increased cell density in the tumor. At low field-strengths, a choline peak is not regularly identified in normal breast tissue [103, 104], and therefore, its presence helps in the differentiation of benign from malignant lesions [105–107]. 1H-MRS can be performed as single-voxel or multi-voxel MRS. Single-voxel spectroscopy provides a single spatially localized spectrum that represents the average chemical signal from a 3D voxel placed in the lesion detected with DCE-MRI. In multi-voxel MRS using chemical shift, a larger volume is excited, producing a spatially resolved grid of spectra. For a detailed review of acquisition techniques and analysis of breast MRS, refer to a recent review article by Bolan et al. [105]. Assessment of the recorded tCho as an indicator of breast malignancy can be performed either qualitatively—detection of the presence of a tCho peak, semi-quantitatively—measurement of tCho SNR, peak height, or peak integral, or as an absolute quantification—calculation of tCho concentration with water as an internal reference or using an external standard. A detrimental effect of contrast agents on 1H-MRS has been described in both experimental and clinical settings, and therefore, the possibility of altered tCho signal intensities after contrast medium injection have to be considered when calculating absolute tCho quantification [103, 105, 108, 109]. Due to the challenging image acquisition and post-processing, the clinical value of proton 1H-MRS remains controversial. Nevertheless, several studies, mainly performed at 1.5 T and using single-voxel approaches, have demonstrated that 1H-MRS can improve diagnostic accuracy in breast cancer diagnosis [10]. In a recent meta-analysis, which included 19 studies, Baltzer et al. [23] evaluated the diagnostic performance and feasibility of 1H-MRS for differentiating malignant and benign breast lesions. The pooled sensitivity and specificity of 1H-MRS was 73 % and 88 %, respectively. There was a substantial heterogeneity of sensitivity in the studies (42–100 %), whereas specificity showed little variation. The meta-analysis did not show any significant performance advantages of 3 T over 1.5 T field strength or multivoxel over single-voxel techniques, or qualitative over quantitative tCho assessments. However the numbers of studies using 3 T (2/19) and MRSI (3/19) were small. 1H-MRS seems to be limited in the diagnosis of early breast cancer and small breast tumors as well as in non-mass-enhancing lesions.
Recently, Gruber et al. [110] developed a high-spatial-resolution 3D 1H-MRSI protocol at 3 T, designed to cover a large fraction of the breast in a clinically acceptable measurement time of 12–15 min with excellent data quality (Fig. 15.3). In that study, with a Cho SNR threshold level of 2.6, 3D 1H-MRSI provided a sensitivity of 97 % and a specificity of 84 % in breast cancer diagnosis.
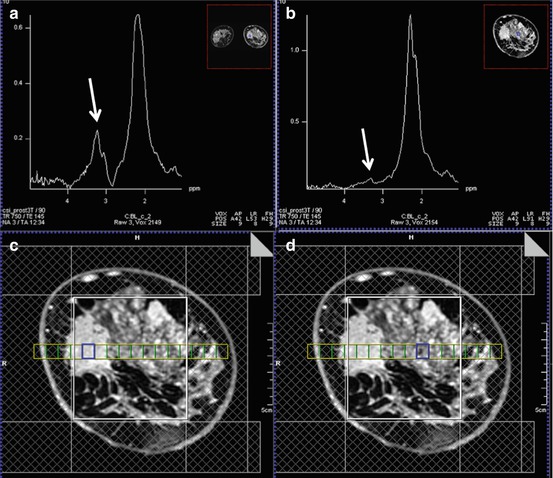
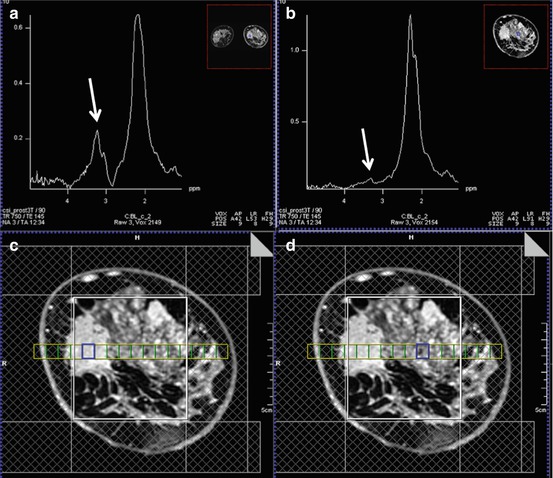
Fig. 15.3
3 T. Invasive ductal carcinoma G3 in a 47-year-old woman at 9 o’clock in the right breast. (a) The spectrum of the voxel with the highest Cho SNR inside the malignant lesion is displayed. An elevated Cho signal at 3.2 ppm was found and the calculated Cho SNR in this voxel was 10.5 (white arrow). (b) The DCE MR images are overlaid with the grid used for 3D 1H-MRSI localization. (c) Metabolic map of (d) adjacent breast tissue without any choline peak (white arrow). DCE-MRI demonstrated an irregularly shaped mass lesion with spiculated margins. DCE-MRI and 3D 1H-MRSI at 3 T concordantly classified the lesion as BI-RADS 5 (highly suggestive of malignancy)
1H-MRSI might also be a valuable tool in the assessment of the response to neoadjuvant chemotherapy [102, 111, 112]. Studies indicate that breast tumor tCho levels and the changes in these levels during treatment are reflective of treatment-induced alterations in cell proliferation prior to any changes in tumor size. 1H-MRSI can, therefore, provide an early predictive imaging biomarker of treatment response. Meisamy et al. demonstrated that MRS of the breast was able to detect a change in Cho concentration from baseline (before receiving chemo) within 24 h of administration of the first dose of the regimen. This change had a statistically significant positive correlation with change in final size (p = 0.001) [104]. In addition, Shin et al. showed that the tCho of tumors was higher in invasive versus in situ cancers and correlated this with several prognostic factors, including nuclear grade, histologic grade, and estrogen receptor (ER) status [106]. Therefore, it can be expected that the addition of 1H-MRSI of the breast will offer a substantial advantage over contrast-enhanced MRI of the breast alone in the prediction of response to neoadjuvant chemotherapy.
In addition, tCho seems to be indicative not only of an increased proliferation, but also a hallmark of imminent malignant transformation [113, 114]. Recently, Ramadan et al. [115] demonstrated that, in BRCA-1 and BRCA-2 carriers, healthy breast tissue is likely to differ from each other as well as from non-mutation carriers with regard to levels of triglycerides, unsaturated fatty acids, and cholesterol in the absence any other imaging findings. Further studies are warranted, but if these findings may be confirmed there might be relevant clinical implications for the screening of high-risk women.
15.2.5 Multiparametric MRI
Available data suggests that the addition of functional MRI parameters, in combination with DCE-MRI, may provide additional specificity [23, 24]. Multiparametric MRI of the breast simultaneously and non-invasively acquires multiple imaging biomarkers, and thus, has the potential to significantly improve breast cancer diagnosis, staging, and assessment of treatment response. Several recent studies have assessed multiparametric MRI using DCE-MRI and DWI for breast cancer diagnosis and have demonstrated that an increased diagnostic accuracy in breast cancer diagnosis could be achieved [116, 117]. To solve the dilemma of how to combine the unique information from DCE-MRI and DWI, and to implement multiparametric MRI in the clinical routine, several different approaches have been explored. Pinker et al. [13] developed a reading scheme that adapted ADC thresholds to the assigned BI-RADS® classification. In that study, the sensitivity of the BI-RADS®-adapted reading was not significantly different from the high sensitivity of DCE-MRI (p = 0.4), whereas the BI-RADS®-adapted reading maximized specificity to 89.4 %, which was significantly higher compared to DCE-MRI alone (p < 0.001). The authors concluded that BI-RADS®-adapted reading, combining DCE-MRI and DWI, improves diagnostic accuracy and is fast and easy to use in routine clinical practice (Fig. 15.4). In a different approach, Baltzer et al. [118] investigated the improvements in specificity of breast MRI by integrating ADC values with DCE-MRI using a simple sum score. The additional integration of ADC scores achieved an improved specificity (92.4 %) compared to DCE-MR-only reading (specificity of 81.8 %), with no false-negative results. Pinker et al. compared the diagnostic accuracy of DCE-MRI as a single parameter to multiparametric MRI with two (DCE-MRI and DWI) and three (DCE-MRI, DWI and 1H-MRSI) parameters in breast cancer diagnosis. Multiparametric MRI with three parameters yielded significantly higher AUCs (0.936) compared to DCE-MRI alone (0.814) (p < 0.001). Multiparametric MRI with just two parameters at 3 T did not yield higher AUCs (0.808) than DCE-MRI alone (0.814). Multiparametric MRI with three parameters resulted in elimination of false-negative lesions and significantly reduced the false-positives (p = 0.002) (Figs. 15.5 and 15.6). The authors concluded that multiparametric MRI with three parameters increases the diagnostic accuracy of breast cancer, compared to DCE-MRI alone and MP MRI with two parameters, and should be considered for future implementation in breast cancer care [11].
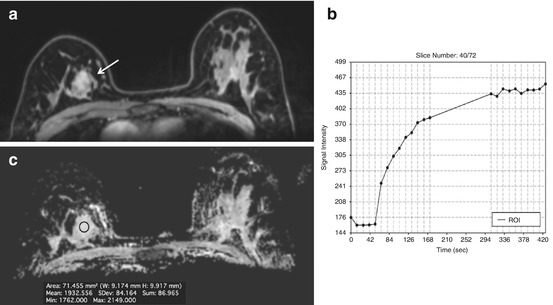
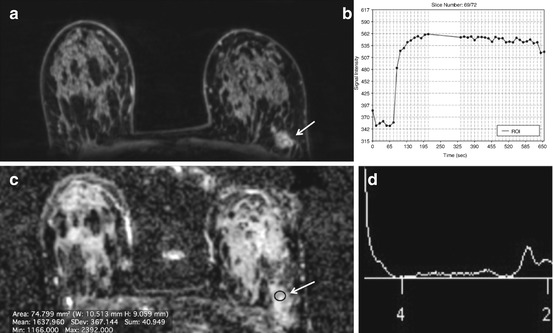
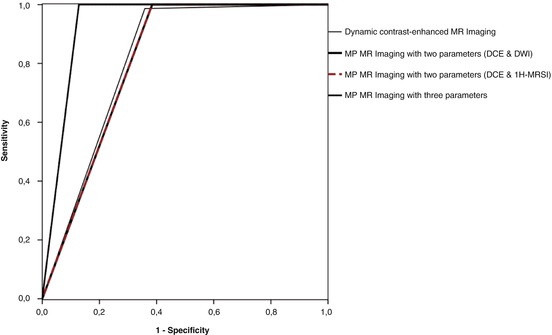
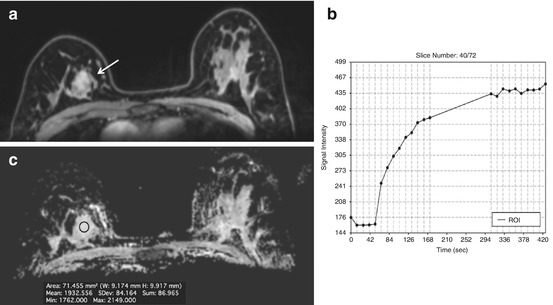
Fig. 15.4
Fibroadenoma in a 48-year-old woman at 2 o’clock in the right breast. (a) The irregular-shaped and partially irregularly marginated mass (white arrow) demonstrated (b) an initial fast/persistent (II) heterogeneous contrast enhancement. (c) However, on diffusion-weighted imaging (DWI), the ADC values (1.93 × 10−3 mm2/s) were well above the threshold for malignancy, allowing an accurate classification as a benign finding with the BI-RADS©-adapted reading
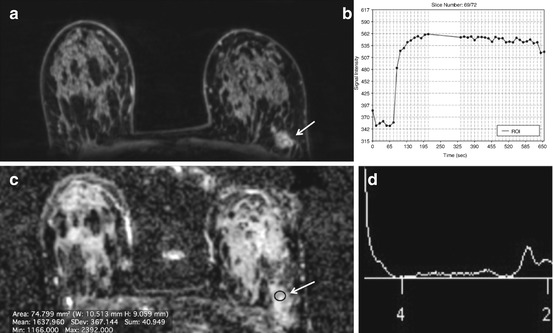
Fig. 15.5
Fibroadenoma in a 48-year-old woman at the 2 o’clock position of the right breast. (a–d) The slightly irregular, partly indistinct mass (arrow) with a heterogeneous initial strong enhancement and plateau curve is classified by DCE-MRI of the breast as BI-RADS© 4 (suspicious abnormality)
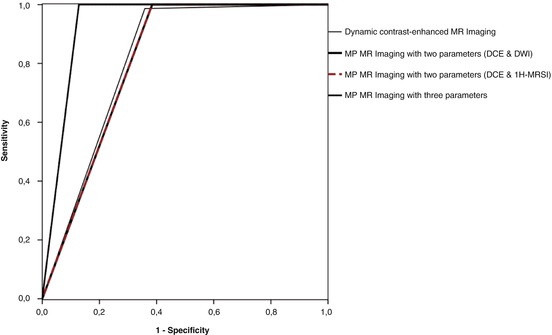
Fig. 15.6
ROC curves illustrate the higher diagnostic value (i.e., higher sensitivity, specificity, and larger area under the curve) that is reached for multiparametric MRI with three parameters, compared to DCE-MRI of the breast and multiparametric MRI with two parameters
Recently, the concept of multiparametric imaging has been extended to ultra-high-field MRI. Pinker et al. evaluated the clinical application of multiparametric MRI using DCE-MRI and DWI at 7 T and its impact on diagnostic accuracy in breast cancer diagnosis [12]. Multiparametric MRI, combining high-resolution DCE-MRI and DWI at 7 T, yielded a sensitivity and specificity of 100 % and 88.2 %, respectively, with an AUC of 0.941, which was significantly greater than DCE-MRI (p = 0.003), with a sensitivity and specificity of 100 % and 53.2 %, respectively, with an AUC of 0.765, and greater than DWI, with a sensitivity and specificity of 93.1 % and 88.2 %, respectively, with an AUC of 0.907 (Fig. 15.7). In that study, multiparametric MRI of the breast at 7 T accurately detected all cancers, reduced false-positives from eight with DCE-MRI to two (Fig. 15.8), and thus, could have obviated unnecessary breast biopsies (p = 0.031). In a very recent study, Schmitz et al. investigated multiparametric MRI with three parameters, i.e., DCE-MRI, DWI, and phosphorus spectroscopy (31P MRSI) at 7 T for the characterization of breast cancer [119]. The authors concluded that multiparametric 7 T breast MRI with three parameters is feasible in the clinical setting and shows an association between ADC and tumor grade, and between 31P MRSI and mitotic count.
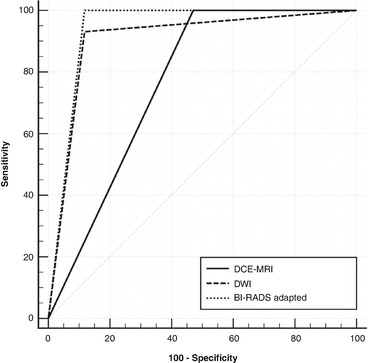
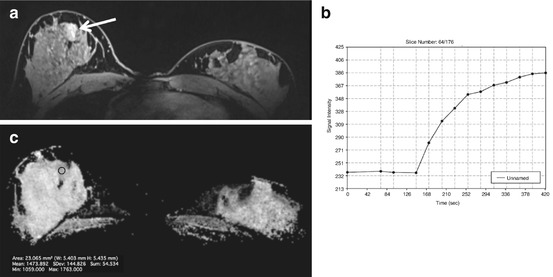
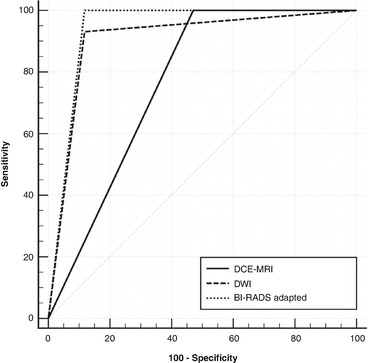
Fig. 15.7
ROC curves illustrate the higher diagnostic value that is reached for multiparametric MRI compared to DCE-MR and DW imaging of the breast at 7 T
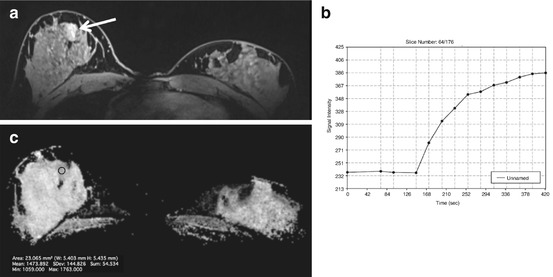
Fig. 15.8
7 T DCE-MR. Fibroadenoma in a 60-year-old woman at 3 o’clock in the right breast. (a) The irregular-shaped and partially irregularly marginated mass (white arrow), demonstrated (b) an initial medium/persistent (II) heterogeneous contrast enhancement on DCE-MRI of the breast at 7 T. (c) However, on diffusion-weighted imaging (DWI), the ADC values (1.47 × 10−3 mm2/s) were well above the threshold for malignancy, allowing an accurate classification as a benign finding with the BI-RADS©-adapted reading
15.3 Emerging MRI Parameters
15.3.1 Sodium Imaging (23Na MRI)
Sodium (23Na) MRI has been introduced as a novel MRI parameter for the detection and therapy monitoring of breast cancer. 23Na MRI provides information about the physiological and biochemical state of tissue, and the sodium concentration is a sensitive indicator of cellular metabolic integrity and ion homeostasis [120, 121]. In normal cells, a low intracellular sodium concentration is maintained by the Na+/K+ ATPase pump, which actively pumps sodium out of the cell against a concentration gradient formed by the higher extracellular sodium concentration. 23Na MRI is able to detect increased sodium levels secondary to failure of the Na+/K+-ATPase pump due to the breakdown of cell membranes, as observed in malignancy. Ouwerkerk et al. investigated the potential of 23Na MRI for the differentiation of benign and malignant breast lesions at 1.5 T [122]. The authors demonstrated that an increased total sodium concentration in breast tumors is a sensitive cellular-level indicator associated with malignancy, and has the potential to increase the specificity of MRI of the breast. However, at field-strengths of 1.5 and 3 T, 23Na MRI is limited. Recently, Zaric et al. investigated quantitative 23Na MRI at 7 T compared to DWI. These authors demonstrated that quantitative 23Na MRI at 7 T can be accomplished with a good resolution and image quality within clinically acceptable measurement times in patients with breast tumors. Quantitative 23Na MRI allowed good discrimination of benign and malignant breast lesions (p = 0.002), similar to DWI (p = 0.002). 23Na MRI reliably provides complementary information about pathophysiologic changes in neoplasms and has the potential to improve the detection, characterization, and treatment monitoring of breast lesions (Fig. 15.9) [123].
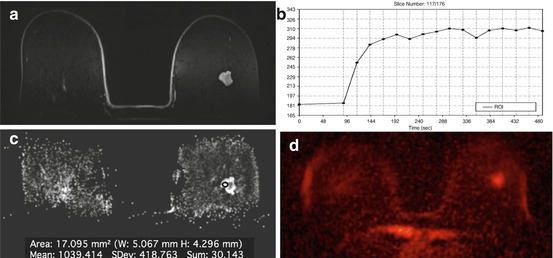
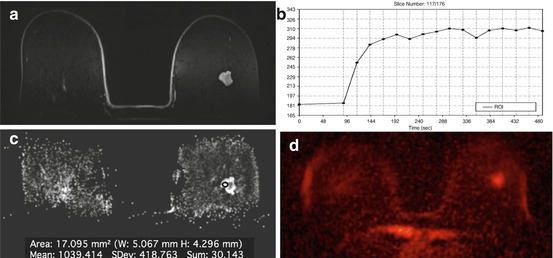
Fig. 15.9
Invasive ductal carcinoma G1 in a 71-year-old woman at 2 o’clock in the right breast. (a) The irregular shaped and partly irregularly marginated mass lesion demonstrates (b) homogeneous initial strong enhancement followed by a plateau. (c) The mass demonstrates low ADC values (1.038 × 10−3 mm2/s) and (d) shows a high signal intensity on 23Na MRI, indicative of malignancy
15.3.2 Phosphorus Spectroscopy (31P MRS)
Phosphorus spectroscopy (31P MRS) measures the bioenergetics of tissue and membrane phospholipid metabolism. The signals of phospholipid precursors and catabolites can be used as imaging biomarkers for tumor progression and response to therapy [124, 125]. It has been demonstrated in several in vitro and in vivo 31P MRS studies that elevated levels of phosphocholine (PC)/phosphoethanolamine (PE) are detectable in several cancers. Barzilai et al. showed a significant decrease in the PE/PC ratio in malignant breast tumors compared to benign lesions [126], as well as changes in PE/PC ratios in response to therapy. At field-strengths of 1.5 and 3 T, the application of 31P MRSI is limited to relatively large and primarily superficial tumors [125]. Recently, the feasibility of 31P MRS at 7 T has been demonstrated in healthy volunteers and patients with breast cancer, with excellent quality of (31P)-MR spectra. Patients with breast cancer show higher levels of PE and PC than healthy volunteers. 31P MRS provides a direct method for the in vivo detection and quantification of endogenous biomarkers, such as phospholipid metabolism, phosphate energy metabolism, and intracellular pH, and allows monitoring in vivo metabolism during neoadjuvant chemotherapy. It can be expected that 31P MRS could used as specific tool for breast cancer diagnosis, tumor staging, and monitoring response to therapy [56, 127].
15.3.3 Chemical Exchange Saturation Transfer (CEST) Imaging
Chemical exchange saturation transfer (CEST) is an MRI parameter that enables visualization of chemical exchange processes between protons bound to solutes and surrounding bulk water molecules [128, 129]. It has been demonstrated that endogenous CEST can discriminate tumor from healthy breast tissue based on the information about protons associated with mobile proteins through the amide proton transfer (APT) effect, and also has been implicated as a prognosticator of response to therapy [130]. Recently, ATP CEST MRI at 3 T has been investigated for its potential in breast cancer diagnosis, with promising results. Schmitt et al. demonstrated lesion detection and differentiation was equally possible with DCE-MRI and ATP CEST-MRI through the CEST contrast generated by endogenous solute molecules. The results of this initial feasibility study hint at a significant potential for ATP CEST-MRI in breast imaging. Recently, animal studies have investigated CEST contrasts other than ATP, exploiting the entire CEST spectrum. Desmond et al. found that imaging of the amide, amine, and aliphatic signal (aaaCEST) allows non-invasive differentiation of areas of apoptosis and/or necrosis from actively progressing tumor [131, 132]. In addition, similar to [18F]Fluorodeoxyglucose positron emission tomography ([18F]FDG), dynamic CEST imaging after the administration of glucose (glucoCEST) has been shown to enable the non-invasive evaluation of the kinetics of glycolysis, which is typically increased in malignant lesions. Initial results indicate that glucoCEST might serve as a potential substitute for PET/CT or PET/MRI in the clinic for the detection of tumors and metastases, distinguishing between malignant and benign tumors and monitoring tumor response to therapy, without the need for radio-labeled isotopes [131, 133–135]. Nevertheless, further studies will be necessary to explore the true potential of CEST imaging in breast cancer.
15.3.4 Blood Oxygen Level–Dependent MRI
Blood oxygen level–dependent (BOLD) MRI or intrinsic susceptibility-weighted imaging is a noninvasive method for the indirect measurement of tumor perfusion and hypoxia. Hypoxia is a feature of most solid tumors, including breast cancer, and is associated with tumor progression, angiogenesis, treatment resistance, local recurrence, and metastasis. BOLD MRI non-invasively detects increased levels of paramagnetic deoxyhemoglobin. Deoxyhemoglobin causes susceptibility variations in the magnetic field, which, in turn, decrease the transverse relaxation rate R2 * ( = 1/T2 *) of water in blood and the tissue surrounding blood vessels. An increase in the deoxyhemoglobin concentration, i.e., hypoxia, causes a decrease in the signal intensity on the T2 * image and a faster R2 * [136]. Improvement in tissue oxygenation has the opposite effect. Therefore deoxyhemoglobin acts as an intrinsic BOLD contrast agent for imaging tissue hypoxia [10, 137]. Initial results indicate that BOLD MRI is a simple and non-invasive technique yielding hypoxia information on breast cancer [91, 138, 139], and can be used to assess response to neoadjuvant treatment [140]. Tumor hypoxia might, therefore, have the potential to serve as an imaging biomarker of breast cancer prognosis as well as treatment response [141]. To date, tumor hypoxia is assessed on biopsy-derived tumor tissue samples, with the main limitations being the invasiveness, non-representative sampling (the tumor can be quite heterogeneous and biopsies can be non-representative of the whole tumor), and the necessity to perform multiple evaluations to follow changes in tumor oxygenation after treatment [141]. These limitations highlight the importance of developing imaging biomarkers that can reliably detect tumor hypoxia for tumor grading and non-invasive monitoring spatio-longitudinally during treatment. However, to date, BOLD MRI in breast imaging is in the experimental/translational stage and data is, as yet, scarce. Further studies will be necessary to elucidate the clinical value of BOLD MRI for breast cancer diagnosis and treatment response assessment.
15.3.5 Hyperpolarized MRI (HP MRI)
Hyperpolarized MRI (HP MRI) is one of the most recent advances in molecular imaging. HP MRI allows a rapid, radiation-free, non-invasive investigation of tumor metabolism by exploiting exogenous contrast agents that have been “hyperpolarized.” Conventional MR imaging depends on nuclear spins that have been polarized on the order of a few parts per million, whereas, in HP MRI, nuclear spins reach near-unity polarization, resulting in an extensive increase in signal intensity [142, 143]. HP MRI nuclear spins are polarized in an amorphous solid state at ~1.2 K through coupling of the nuclear spins with unpaired electrons that are added to the sample via an organic free radical. In the solid state, the high electron spin polarization is, in part, transferred to the nuclear spins by microwave irradiation and then the sample is rapidly dissolved for injection. Recently, 13C-labeled substrates have been polarized to obtain enhancements of the 13C nuclear MR signals, e.g., >50,000-fold at 3 T in the substrate as well as subsequent metabolic products [144]. The enhancement that is achieved is lost in time as a function of the spin-lattice relaxation time of the nucleus (T1). The HP 13C probes can be injected into living organisms and their metabolism can be observed in real-time by chemical shift imaging. Currently, (13C) pyruvate is the most widely used probe for HP MR studies since it polarizes well, has a long T1 relaxation time, and is rapidly taken up by the cell and metabolized at the juncture of glycolysis, TCA, amino acid biosynthesis, and other critical pathways. Multiple animal studies have confirmed that the real-time measurement of the relative transformation of pyruvate into lactate and alanine, using HP MRI, allows a differentiation of benign and malignant tissue [145–147]. In addition to the distinction of cancerous and normal cells [146, 148, 149], HP MRSI using 13C pyruvate has been demonstrated to have potential in the assessment of cancer progression [150, 151]. Recently, other novel probes for redox (13C dehydroascorbate), necrosis (13C fumarate), and glutamine metabolism (13C glutamine) have been developed to interrogate other metabolic pathways, with promising results [152]. To date, there is no specific clinical application for HP MRI in breast cancer. Nevertheless, several pre-clinical and initial studies in cancer, including breast cancer [153], indicated that this technique may be applicable for the detection of breast cancer and assessment of treatment response in the future.
15.4 Nuclear Imaging of the Breast
15.4.1 Gamma Camera Imaging: 99mtc-Sestamibi Scintimammography (SM) and Breast-Specific Gamma Imaging (BSGI)
99mTc-sestamibi scintigraphic mammography (99mTc-MIBI-SM) was introduced in the ’90s and can be used as an alternative diagnostic imaging modality in patients with dense breasts. In 99mTc-MIBI-SM, the radiotracer 99mTc-MIBI is injected intravenously and accumulates in tissues with increased perfusion, permeability, and metabolic activity, such as breast cancer. Several studies have investigated the application of 99mTc-MIBI for the assessment of breast tumors, using both planar and single-photon emission computed tomographic radionuclide imaging with a general-purpose gamma camera, and have reported sensitivities ranging from 84 to 93 % [154]. However, 99mTc-MIBI-SM has a relatively low spatial resolution, which impedes the depiction of small cancers and is limited in the detection of low-grade breast tumors [155–157]. To overcome these limitations, 99mTc-MIBI-SM Breast-Specific Gamma Imaging (BSGI) has been developed [158–163]. In a meta-analysis, Sun et al. reviewed the role of BSGI as an adjunct modality to mammography [164] and reported a pooled sensitivity and specificity of 95 % and 80 %, respectively. In patients with normal mammography findings, 4 % were diagnosed with breast cancer by BSGI, and, in those with a suspicious imaging finding on mammography or biopsy-proven breast cancer, 6 % were diagnosed with multifocal disease by BSGI. The authors concluded that BSGI can serve as a valuable adjunct modality to mammography for detecting breast cancer.
15.4.2 Positron Emission Tomography (PET)/Computed Tomography (CT), and PET Mammography (PEM)
Positron emission tomography (PET) is a non-invasive diagnostic nuclear medicine imaging method that enables the assessment of physiological processes using radiotracers. The most commonly used radiotracer is [18F]Fluorodeoxyglucose ([18F]FDG). [18F]FDG PET allows an assessment of tissue glycolysis, which is typically increased in neoplastic processes, such as breast cancer [165–167]. However, [18F]FDG uptake is variable based on the organ of origin, tumor type, and grade, and a critical mass of tumor cells is necessary for visualization. In addition, [18F]FDG uptake is not tumor-specific and some benign conditions, such as inflammatory processes, can also be [18F]FDG-avid. As [18F]FDG PET alone provides limited anatomical information and has a low spatial resolution that frequently results in difficulties in lesion localization and in the assessment of potential tumor infiltration into adjacent organs, hybrid imaging systems, such as PET/CT, were developed and established in the clinical routine. Numerous studies have investigated [18F]FDG PET and [18F]FDG PET/CT, in the supine position, for breast imaging [165–170]. 18FDG-PET/(CT) is helpful in the staging of locally advanced metastatic or recurrent breast cancer, as well as in evaluating the response of locally advanced and metastatic breast cancer to treatment. Available data indicate that ([18F]FDG PET/CT is valuable in the evaluation of advanced axillary disease and nodal spread in locally advanced breast cancer [165–171]. Whole-body supine [18F]FDG PET/CT has been evaluated for the detection and staging of primary breast cancer with reported sensitivities and specificities of 88 % and 80 %, respectively. However, due to its limited ability in the detection of small lesions and low grade cancers, it is currently not recommended for local staging of known or suspected primary breast malignancies [172, 173]. In a recent study, Magometschnigg et al. compared the diagnostic accuracy of [18F]FDG PET/CT with DCE-MRI at 3 T in suspicious breast lesions, evaluated the influence of tumor size on diagnostic accuracy, and explored the use of SUVMAX thresholds to differentiate malignant and benign breast lesions [173]. In that study, the patients were scanned in the prone position, with a state-of-the-art combined PET/CT system, which might explain the achieved higher sensitivity and diagnostic accuracy. Both [18F]FDG PET/CT and DCE-MRI demonstrate an equal diagnostic accuracy for breast cancer diagnosis of 93 % (Fig. 15.10). Neither sensitivity (p = 0.125), specificity (p = 0.344), or diagnostic accuracy (p = 1) were significantly different. In lesions <10 mm, diagnostic accuracy deteriorated to 91 % for both [18F]FDG PET/CT and DCE-MRI. In lesions <10 mm, CE-MRI at 3 T is more sensitive, but less specific than [18F]FDG PET/CT. Quantitative assessment using an SUVMAX threshold for the differentiation of benign and malignant lesions is not helpful in breast cancer diagnosis.
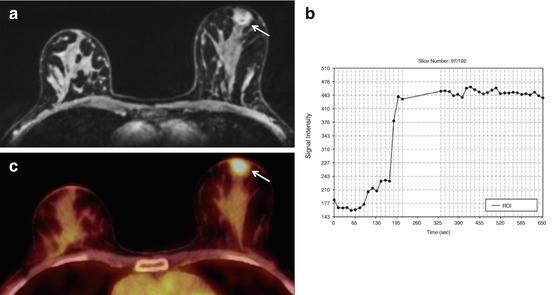
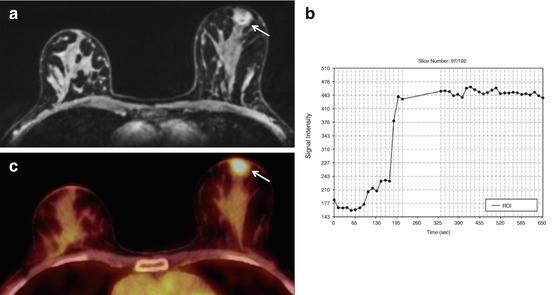
Fig. 15.10
Invasive ductal carcinoma G2 in a 46-year-old woman, retroareolar in the right breast. (a) The round, irregularly marginated mass lesion (white arrow) shows (b) a heterogeneous initial strong enhancement followed by a plateau, and was classified by CE-MRI as BI-RADS© 5 (suspicious finding). (c) On 18F-FDG PET/CT, the lesion was strongly 18F-FDG-avid, with an SUVMAX of 4.4. The lesion was a true-positive finding on both modalities
The authors concluded that [18F]FDG PET/CT can be considered an alternative imaging modality in patients who are not candidates for DCE-MRI.
To overcome the limitations of whole-body [18F]FDG PET/CT in breast imaging, dedicated breast PET systems have been developed. Positron Emission Mammography (PEM) is a high-resolution, breast-specific device that enables co-registration of mammographic and emission [18F]FDG images of the breast by means of two flat detectors integrated into the system on either side of the breast. The more recently developed breast-specific MAMography with MolecularImaging (MAMMI)-PET utilizes a small ring of detectors, which yields an improved contrast and signal-to-noise ratio (SNR). Other alternatives to conventional PEM design are the Clear-PEM system or the Shimadzu, which acquire tomographic breast images. Dedicated breast PET systems have been shown to have both a high sensitivity and specificity in detecting breast malignancy (<1 cm). Kalles et al. reviewed the role of [18F]FDG PEM in breast cancer imaging, and concluded that [18F]FDG PEM can successfully complement conventional imaging in breast cancer detection by providing information about tumor biology. The current data suggest that PEM might not be far from being included in the first-line modalities for breast cancer screening [174].
15.5 Molecular Imaging with PET/MRI
In their multi-step development, cancers have acquired several biological capabilities, such as sustaining proliferative signaling, evading growth suppressors, resisting cell death, enabling replicative immortality, inducing angiogenesis,s and activating invasion and metastasis [175–179]. To elucidate these hallmarks of cancer, imaging techniques have to be equally sophisticated and multilayered. Both MP MRI and PET of the breast can visualize different processes involved in cancer development and progression, thus providing morphologic, functional, metabolic, and molecular information about breast tumors. To overcome the individual limitations of morphologic and functional imaging techniques, hybrid imaging systems have been developed and introduced into the clinical routine. Initial studies investigating fused [18F]FDG PET and DCE-MRI for breast cancer diagnosis demonstrated that fused [18F]FDG PET/MRI provides accurate morphological and functional data and has the potential to emerge as an all-encompassing alternative to conventional multi-technique tumor staging [180–183]. However, in these studies, the functional information provided by [18F]FDG PET was merely combined with the morphologic and limited functional information of DCE-MRI. Pinker et al. investigated multiparametric PET/MRI using DCE-MRI, DWI, 1H-MRSI, and 18FDG for the assessment of breast tumors at 3 T [184]. The authors demonstrated that MP [18F]FDG PET/MRI provided an improved differentiation of benign and malignant breast tumors when several MRI and PET parameters are combined. In addition, the authors concluded that MP [18F]FDG PET/MRI may lead to a reduction of unnecessary breast biopsies of up to 50 % (Fig. 15.11). In a recent feasibility study, Pinker et al. investigated combined PET/MRI of breast tumors in eight patients with DCE-MRI, DWI, the radiotracer [18F]FDG, and the hypoxia tracer [18F]FMISO (refer to Sect. 15.5.1) at 3 T (Fig. 15.12), and correlated MRI and PET parameters with pathological features, grading, proliferation-rate (ki67), immuno-histochemistry, and the clinical endpoints metastasis and death. Preliminary results showed several moderate to excellent correlations between quantitative imaging markers, grading, receptor status, and proliferation rate (Fig. 15.13) [185]. Multiparametric criteria provided independent information. DCE-MRI, [18F]FDG- and [18F]FMISO-avidity strongly correlated with the presence of metastasis [r = 0.75 (p < 0.01), 0.63 (p = 0.212), and 0.58 (p = 0.093)] and death [r = 0.60 (p = 0.09), 0.62 (p = 0.08), 0.56 (p = 0.11)]. Multiparametric [18F]FDG /[18F]FMISO PET/MRI provides quantitative prognostic information in breast cancer patients, and thus, might have the potential to enable tailored therapy through improved risk stratification.
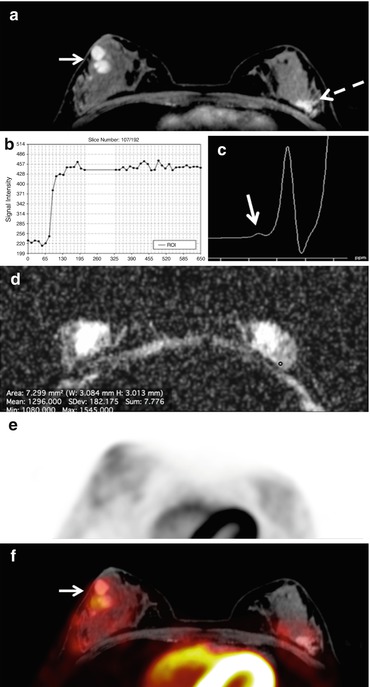
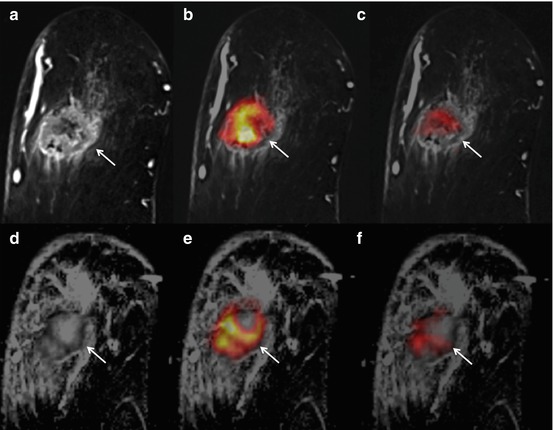
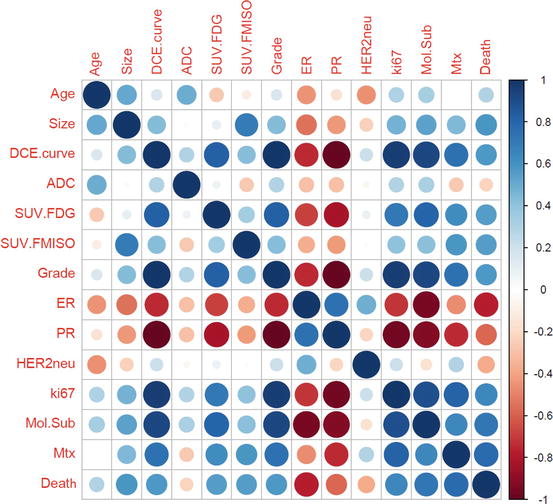
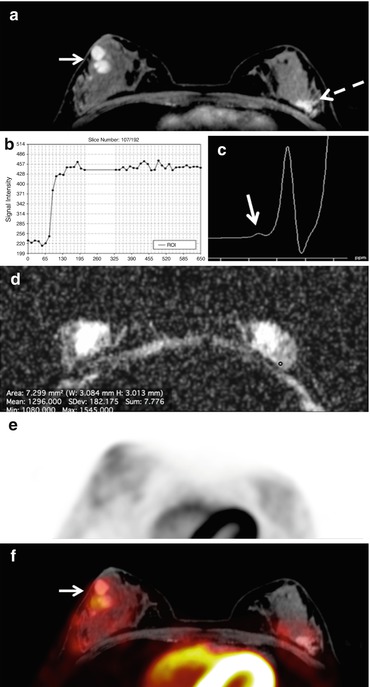
Fig. 15.11
PET/MRI. Fibroadenomatous hyperplasia in a 22-year-old woman in the upper outer quadrant of the left breast. (a) The irregular shaped and partly irregularly marginated mass lesion (white dashed arrow) demonstrates (b) homogeneous initial strong enhancement followed by a plateau. (c) However, the ADC values are not below the cut-off for malignancy (1.275 × 10−3 mm2/s). (d) There is no Cho peak depicted at 3.23 ppm in 3D 1H-MRSI (arrow) and (e) the lesion is not [18F]FDG-avid. The lesion is false-positive on DCE-MRI, but true-negative on (f) multiparametric PET/MRI. Note two classic, mildly metabolically active fibroadenomas in the right breast, retroareolar (white arrow)
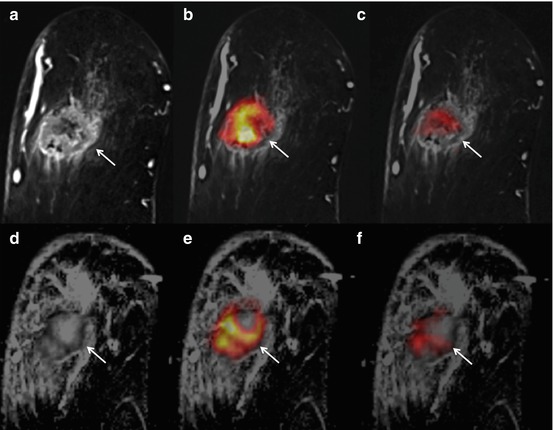
Fig. 15.12
Invasive ductal carcinoma G3 in a 55-year-old woman at 9 o’clock in the left breast. Upper row: DCE and fused [18F]FDG and [18F]FMISO PET/MRI. Lower row: ADC and fused [18F]FDG and [18F]FMISO PET/MRI. (a) The indistinct irregular mass lesion (white arrow) with heterogeneous enhancement shows decreased (d) ADC values in the enhancing areas. The lesion is (b, e) highly [18F]FDG-avid and (c, f) several tumor areas show [18F]FMISO-avidity indicative of tumor hypoxia
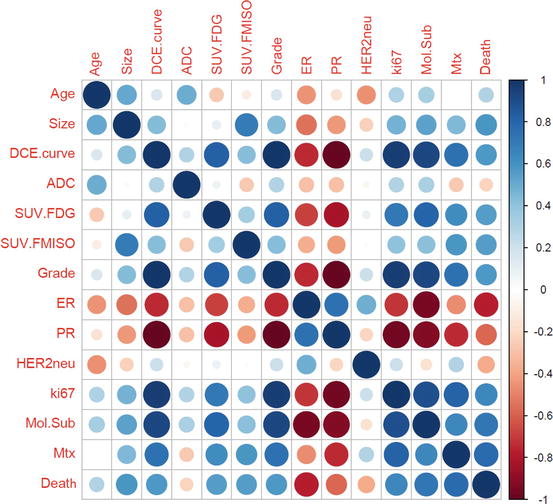
Fig. 15.13
Non-parametric Pearson’s correlation coefficient matrix. Correlations are marked in blue if positive and red if negative (cf. legend on the left) DCE dynamic contrast enhanced-MRI, ADC apparent diffusion coefficient, SUV standardized uptake value, FDG fluorodeoxyglucose, FMISO Fluorine-18 labeled misonidazole, ER estrogen receptor, PR progesteron receptor, HER 2 NEU human epidermal growth factor receptor 2, Mol.Sub molecular subtype, Mtx metastases
15.5.1 Specific Radiotracers
To date, nuclear and hybrid imaging in breast cancer is mainly performed using the radiotracer [18F]FDG. Although [18F]FDG is a very sensitive radiotracer, it is limited with regard to specificity, as there is a significant overlap in uptake behavior for benign and malignant conditions. Recently, more specific radiotracers to target processes involved in cancer development and progression have been developed and introduced into breast imaging:
[18F]Fluoromisonidazole ([18F]FMISO) for the assessment of tumor hypoxia; radioactive-labeled Annexin V for the assessment of tumor-neoangiogenesis; [18F]Fluoro-L-thymidine ([18F]FLT) for the assessment of nucleic acid metabolism; and [18F]Fluoroestradiol ([18F]FES) and radiolabeled trastzumab for the assessment of tumor receptor status.
Tumor hypoxia
[18F]FMISO has a high affinity to hypoxic cells with active nitroreductase enzymes and accumulates in activated tumor cells, but not necrotic cells. Therefore, [18F]FMISO has the potential to serve as an imaging biomarker for tumor grading and assessment of treatment response. Cheng et al. [186] investigated whether [18F]FMISO PET/CT can predict primary resistance to endocrine therapy in estrogen-receptor-positive breast cancer, and found a significantly positive correlation between baseline [18F]FMISO uptake and clinical outcomes after ≥3 months of primary endocrine therapy with letrozole. The data suggest that [18F]FMISO PET/CT may be an effective method for monitoring the response to endocrine therapy, and has the potential for early identification of non-responders.
Apopotosis
Apoptosis plays an important role in tumorgenesis, progression, and therapy. Apopotosis induces a cascade of enzymatic processes, which eventually lead to cell death. The activation of caspsases enables the externalization of phosphatidylserine (PS), which is usually located on the inside of the cell membrane. The protein Annexin V binds to PS with a high affinity, and therefore, is a marker for apoptosis. To date, Annexin V has been labeled with multiple radiotracers for SPECT and PET imaging [187].
Proliferation
The radiotracer [18F]FLT accumulates in proliferating cells. The accumulation is regulated by the thymidine salvage pathway and by the activity of thymidine kinase one, and is, therefore, reflective of DNA synthesis. [18F]FLT has demonstrated promising results for the detection of treatment response in preclinical breast cancer mouse models, and is now under investigation in several clinical trials [188].
Receptor status
[18F]FES PET imaging allows a non-invasive visualization and quantification of estrogen receptor expression of both the primary tumor and metastases [189]. In addition, [18F]FES PET imaging provides valuable information about the response to endocrine therapy, both in the neo-adjuvant and adjuvant setting. In a recent publication by van Kruchten et al., the authors provided a comprehensive overview of the role of [18F]FES PET/CT in breast cancer [190], and concluded that [18F]FES PET/CT has the potential to significantly influence patient management.
Radiolabeled trastuzumab allows the non-invasive visualization and quantification of human epidermal growth factor receptor 2 (HER2) status. In initial studies, Smith-Jones et al. demonstrated the non-invasive measurement of HER2 expression and therapy-induced changes, using a 68Gallium-labeled fragment of trastuzumab in an animal model [191, 192]. In recent clinical PET/CT studies with 64Copper (64Cu)-DOTA-labeled trastuzumab, it was demonstrated that 64Cu-DOTA-trastuzumab PET/CT enables the detection of the primary tumor, as well metastases, with excellent sensitivity, and therefore, has the potential to further improve HER2-targeted therapies [193, 194].
15.6 Conclusion
Within the last few years, multiparametric imaging has entered the field of breast imaging. Multiparametric imaging of the breast comprises established and advanced MRI parameters, such as DWI and MRS, nuclear imaging with PET, PEM, and BSGI, and, more recently, combinations of techniques (e.g., PET/CT and PET/MRI). In addition, novel MRI parameters, such as 23Na-MRI, 31P MRSI, CEST, BOLD, and hyperpolarized MRI, are rapidly evolving and specific radiotracers are being investigated. Multiparametric imaging of the breast is a still-evolving field and more significant advances are imminent. It can be expected that multiparametric imaging of the breast, using cutting-edge sequences and targeted radiotracers, simultaneously at different levels, will provide information, noninvasively, about the hallmarks of cancer. Therefore, multiparametric imaging of the breast has the potential to significantly enhance our understanding of tumor molecular biology and to enable the development of novel personalized approaches in the management of breast cancers thereby significantly impacting not only cancer research, but also clinical practice.
References
1.
DeSantis CE, Bray F, Ferlay J, et al. International variation in female breast cancer incidence and mortality rates. Cancer Epidemiol Biomarkers Prev. 2015. doi:10.1158/1055-9965.EPI-15-0535.PubMed
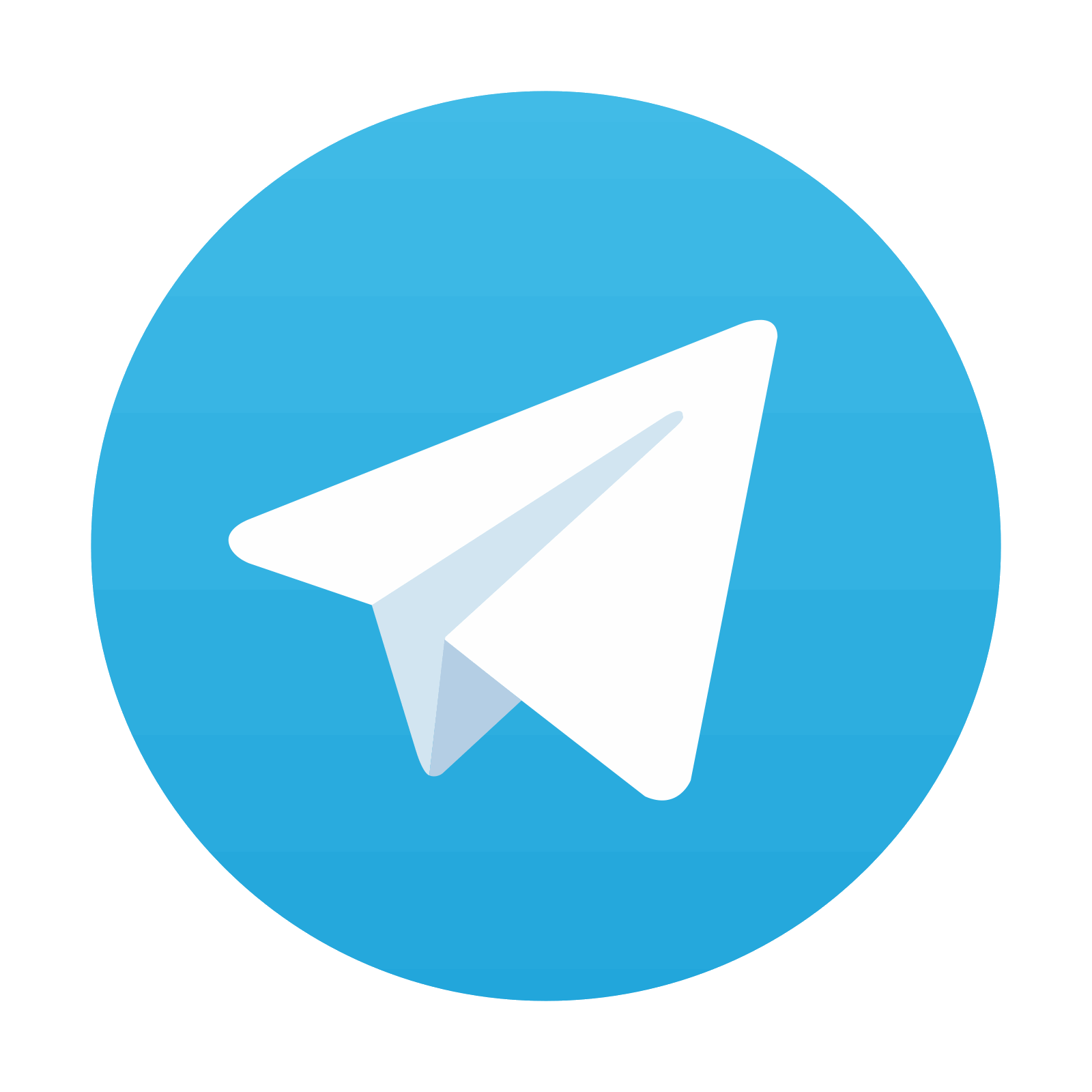
Stay updated, free articles. Join our Telegram channel
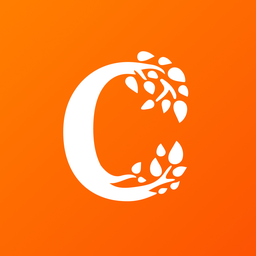
Full access? Get Clinical Tree
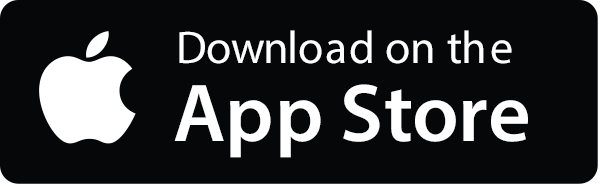
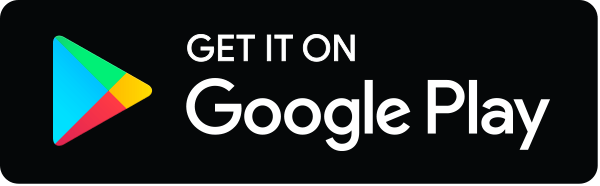