M.A. Hayat (ed.)Stem Cells and Cancer Stem CellsStem Cells and Cancer Stem Cells, Volume 122014Therapeutic Applications in Disease and Injury10.1007/978-94-017-8032-2_4
© Springer Science+Business Media Dordrecht 2014
4. Molecular Mechanisms Underlying Human Somatic Cell Reprogramming to Generate Induced Pluripotent Stem Cells
(1)
Global Science & Innovation Office, R&D, Life Technologies, 5781 Van Allen Way, Carlsbad, CA 92008, USA
Abstract
The discovery by Yamanaka and Thomson has opened a “new era” for biology and regenerative medicine. They showed that by expressing four transcription factors in somatic cells, these cells can be reprogrammed to induced pluripotent stem cells (iPSCs) similar to embryonic stem cells and can give rise to almost every cell type in the human body. The creation of these special cells was major ground-breaking work in cell biology and opened the path for providing unprecedented access to patient-specific iPS cells for drug screening, disease modeling and cell therapy applications. Beside therapeutic issues, iPS cell technology opens the door for broader research on human pluripotent cells because ethical limitations are lifted with iPS cells as compared to hES cells. Therefore, it is not surprising that the methods for generating iPSCs have significantly evolved over the past few years. We are now able to convert essentially any somatic cell type into iPSCs with increased efficiency and at higher quality when compared to ESCs. Despite these advances, the molecular events occurring during various stages of reprogramming remain largely unknown. In this review we will discuss the current understanding of molecular mechanisms underlying human somatic cell reprogramming to generate induced pluripotent stem cells.
Introduction
The first mouse embryonic stem cell line, established in the 1980s, demonstrated their ability to proliferate indefinitely and differentiate into three germ layers. Since then, embryonic stem cells have shown great promise for advancing the fields of drug discovery, disease modeling and regenerative medicine. In the past, it was widely believed that once stem cells differentiated, they could not revert back to an earlier development stage until the invention of somatic cell nuclear transfer (SCNT) (Briggs and King 1952). This technique replaces the nuclei of oocytes with those of somatic cells, resulting in the reversal of the fused cells from a differentiated to pluripotent status. Subsequently, many species of mammalian cells have been successfully cloned, including the famous cloned sheep “Dolly” (Campbell et al. 1996). However, this approach has low success rates and raises ethical issues regarding the use of embryos.
In 2006, Takahashi and Yamanaka successfully generated induced-pluripotent stem cells (iPSCs) with the novel approach of using viral integration of four transcription factors, Oct4, Sox2, Klf4 and cMyc. Subsequently, Yu et al. (2007) also successfully generated iPSCs using another set of four defined factors, Oct4, Sox2, Nanog and Lin28. Induced pluripotent stem cells share many features with embryonic stem cells in that they show similar gene and protein expression profiles and can generate viable, fertile live-born mice (Takahashi and Yamanaka 2006). This revolutionary technique has demonstrated the feasibility of using somatic cells to generate iPSCs. Patient specific iPSCs can now be derived from the cells of the same patient, thereby conceivably avoiding the immune rejection that occurs with SCNT or human embryonic stem cells (hESCs). In addition, the use of somatic cells as the starting material circumvents the ethical issues associated with the use of human embryos. Subsequently, many iPSCs have been generated from various types of somatic cells, including keratinocytes, neuronal stem cells and fibroblasts (Aasen et al. 2008) (Takahashi et al. 2007). These iPSCs display gene expression patterns, cell morphology and the capacity of forming teratoma in vivo similar to those of hESCS (Takahashi et al. 2007). The potential of iPS cell technology shows great promise to the research and medical communities. However, the actual molecular mechanism of induced reprogramming still remains undefined. In this chapter, we will summarize recent advances in iPS cells research and describe some of the molecular processes underlying pluripotency reprogramming.
Mechanisms of Transcription Factors Induce Pluripotency
The original discovery of Tahakashi and Yamanaka (2006) reported the use of four transcription factors Oct4, Sox2, Klf4 and cMyc (OSKM), to reprogram somatic cells to induced pluripotent stem cells (iPSCs). This combination of factors emerged from an initial screen of mouse fibroblasts based on co-transduction of 24 candidate genes (Takahashi and Yamanaka 2006). The generation of iPSCs with this method has now been reported from mouse and human somatic cells using various methods (Carey et al. 2011). Direct reprogramming is a slow and inefficient process, with estimated efficiencies in human cells ranging from 0.02 to 1% (Chin et al. 2009). Studies demonstrated that equal stoichiometry of all four reprogramming factor expressions is a critical contributing factor to successful hiPSC induction (Tiemann et al. 2011). In addition, the expression of four factors is required for a minimum of 12–16 days before activation of pluripotent markers emerge (Ruff et al. 2012).
It is thought that each reprogramming factor plays a distinct role in inducing reprogramming and maintaining pluripotency. The transcription factors Oct4, Sox2 and Nanog have essential roles in early development and are required for maintenance of embryonic stem cells (Boyer et al. 2005). Studies reported that Oct, Sox and Nanog collaborate to form regulatory circuitry consisting of autoregulatory and feedforward loops (Boyer et al. 2005). These factors occupy genes that encode important developmentally homeodomain transcription factors as well as chromatin regulators in ES cells. In addition, it was shown that at the transcriptional level, Oct4 and Sox2 downregulate snail mRNA, the epithelial to mesenchymal transition regulator, during reprogramming (Li et al. 2010). Meanwhile, Klf4 induced E-cadherin mRNA and other epithelial markers (Li et al. 2010), while cMyc reduced TGFb signaling by repressing the TGFb1 and TGFb receptors (Kim et al. 2010). The target genes of these transcription factors are similar in iPSCs to those previously defined in ESCs (Plath and Lowry 2011).
cMyc has been shown to be dispensable for reprogramming, however, with dramatically reduced efficiency and kinetics (Nakagawa et al. 2008). Studies found that cMyc network is largely independent of the core ES cell pluripotency network and thus not involved in the upregulation of the pluripotency network during the final step of reprogramming (Plath and Lowry 2011). Rather, functions of cMyc include targeting genes that are involved in cell proliferation, metabolism, and biosynthetic pathways. A recent report also suggests that cMyc plays a major role in the release of promoter-proximal pausing of RNA polymerase II (Pol II) and thereby enhances the elongation of transcripts, rather than Pol II recruitment at its target genes (Rahl et al. 2010). These suggest that cMyc could enhance, but are not absolutely required for transcription of its target genes. Even though cMyc has been shown to be dispensable for reprogramming, cMyc over expression may lay the framework for the efficient induction of proliferation.
Although partially reprogrammed cells have successfully acquired a proliferative capacity, many pluripotency-related genes are not occupied by Oct4, Sox2 and Klf4, and the endogenous Nanog and Oct4 are not reactivated (Kim et al. 2010). One hypothesis is that in partially reprogrammed cells these transcription factors may need other proteins that are not yet available in pre-iPSCs to allow cooperative binding of the factors to promoter regions. One candidate is the transcription factor Nanog, which has extensive protein-protein interaction and co-localizes with Oct4 and Sox2 at a set of promoter regions in ES cells (Chen et al. 2008). In pre-iPSCs , many of the Nanog target genes completely lack binding of transcription factors as compared to ES cells. Nanog is absolutely essential for the generation of iPSCs, but required only during the final step of reprogramming (Theunissen et al. 2011).
Roles of miRNAs in Reprogramming
In 2004, Suh and colleagues identified a set of embryonic stem cell-specific miRNA which consequently have been found to contribute to embryo development. Moreover, deficiency in these miRNAs can cause detrimental defects in cell proliferation and differentiation. Among the highly expressed ESC-specific miRNAs, miR302/367 is highly expressed in early embryonic development and rapidly declines after differentiation (Ren et al. 2009). Several laboratories have reported the role of miR-302/367 in reprogramming (Subramanyam et al. 2011).
In 2008, Lin et al. and their peers demonstrated that a small noncoding RNA, called miR302, can replace all previously defined factors to reprogram human and mouse somatic cells to ESC-like iPSCs. Elevated miR302 expression triggers both global demethylation and coexpression of Oct4, Sox2 and Nanog in human iPSCs (Lin et al. 2011). MiR-302 is a 23 ribonucleotide microRNA expressed abundantly in human ESCs but is absent in all differentiated tissue cells (Kim et al. 2010). Specifically, it functions as a gene silencer and simultaneously downregulates multiple epigenetic regulators, including lysine specific histone demethylases 1 and 2 (namely AOF2/1, LSD1/2, or KDM1/1B), DNA cytosine 5-methyltransferase 1 (DNMT1) and methyl-CpG binding proteins 1 and 2 (MECP1/2) (Lin et al. 2011). DNA methyltransferase 1 (DNMT1), an essential regulator in DNA methylation, is then silenced in response to the down-regulation of AOF2, leading to genome-wide demethylation and consequently coactivation of pluripotency-promoting genes (Lin et al. 2011). Silencing of these epigenetic regulators induces global DNA demethylation, the first sign of somatic cell reprogramming.
In addition, miR302/367 also directly targets NR2F2, a member of the nuclear orphan receptor family of transcriptional factors and a negative regulator of Oct4 (Rosa and Brivanlou 2011). In hESCs, NR2F2 expression begins with differentiation and conversely correlates with the expression of Oct4 and miR302/367. Studies have also shown that Oct4, Nanog and Sox 2 bind to the promoter regions of miR302/367 and activate miR302 expression level (Marson et al. 2008). Through silencing of AOF1/2, MECP1/2 and DNMT1, we now understand that miR302 induces global demethylation and leads to Oct-4-Sox2-Nanog activation (Lee et al. 2002). The mutual stimulation between miR302 and Oct-Sox-Nanog forms a positive feedback regulation loop to maintain the pluripotent status of reprogrammed iPSCs. In addition, the expression of Lin28 and many other ESC marker genes was observed 1–3 days later, after the presence of Oct4-Sox2-Nanog elevation (Kuo and Ying 2012). The key to somatic cell reprogramming (SCR) is global demethylation, which unlocks and resets these differentiated gene expression patterns to a highly uniform ESC-like profile. This “unlocking” of a genome allows transcription machinery to access to the ESC specific genes and is required for iPSC formation.
To date, viral transfection has been the primary method of introducing four reprogramming factors into cells because of its high efficiency of delivery. However, this method is less ideal for two reasons. One is the possible viral integration of exogenous genes into the host genome. Second, in cases where non-integration viral delivery is used, cMyc and Klf4 are less ideal because both are potential oncogenes. Thus, these factors will affect the safety use of cells in clinical trials. If miRNAs can induce reprogramming events similar to those Yamanaka factors, they will provide a simple and safer way to generate iPSCs due to the fact that no oncogene is required. In addition, the dual function of miR302 in both reprogramming as well as tumor suppression will provide a convenient means to control the quality of iPSCs that are more ideal for human use.
Global Epigenetic Changes in Pluripotent Stem Cells
Previous studies with polycistronic cassettes that encode all four factors indicated that although most of the cells express all four reprogramming factors, only a small subset of cells gets converted to the pluripotent state, resulting in low efficiency between 0.02 and 1% (Jaenisch and Young 2008). Most cells expressing the reprogramming factors fail to successfully induce the first morphological change of proper reprogramming events, remain fibroblast-like and often undergo apoptosis, senescence or cell cycle arrest. Each of these processes is thought to be a barrier to reprogramming and suppressions of these responses result in higher reprogramming efficiency (Kawamura et al. 2009). For example, deletion of p53 and p21 or Ink4a/Arf was found to enhance the efficiency and kinetics of iPSC reprogramming (Kawamura et al. 2009). It is likely that promotion of cell proliferation through S-phase results in resetting chromatin landscapes and improves reprogramming (Ruiz et al. 2011). However, it is still unclear why the majority of cells failed to reprogram completely compared to the number of cells that are proliferative. Only a subset, approximately 3–5%, of the somatic cells that initially express the reprogramming factors eventually convert to the pluripotent state within this time frame. These findings indicate that induction of the pluripotent state requires additional mechanism to drive cells into fully pluripotent state.
Additional reports also suggest that the general reprogramming timeline may require secondary or stochastic events through which certain cells acquire unique advantages that permit transition to pluripotency (Hanna et al. 2009). Thus, the ectopic expression of the current set of embryonic factors appears insufficient to reset the somatic nucleus along, and the mechanism of action likely includes the activation of additional, yet unidentified downstream effectors.
It is currently believed that repressive chromatin comprises a major mechanistic barrier to transcription factor-induced reprogramming. Recent large-scale analyses of DNA methylation and histone modifications revealed dynamic chromatin states and DNA methylation status at promoters and most CpG islands (Meissner et al. 2008), showing that the methylation state of H3K4 is a good indicator of promoter DNA methylation levels in mammalian cells. DNA methylation is a critical component of the epigenome that represses gene expression through promoter CG methylation, in addition to localization at heterochromatin and repetitive elements in the genome. This is consistent with prior studies indicating that H3K4 methylation disrupts DNA methylation by inhibiting contact of DNMTs with histones (Meissner et al. 2008).
Additional studies demonstrate that epigenomic landscape in hESC and lineage committed cells are drastically different and mainly differ in chromatin structure. Most changes arise from dramatic redistribution of repressive H3K9me3 and H3K27me3 marks (Harris et al. 2010). A large number of potential regulatory sequences also exhibit a high degree of dynamics in chromatin modifications and DNA methylation. This is mainly suggested by the ability of agents such as histone deacetylases, histone methyltransferases and demethylases, as well as DNA methyltransferase 1 inhibitors that liberate repressive chromatin states to enhance the process of reprogramming (Mikkelsen et al. 2007).
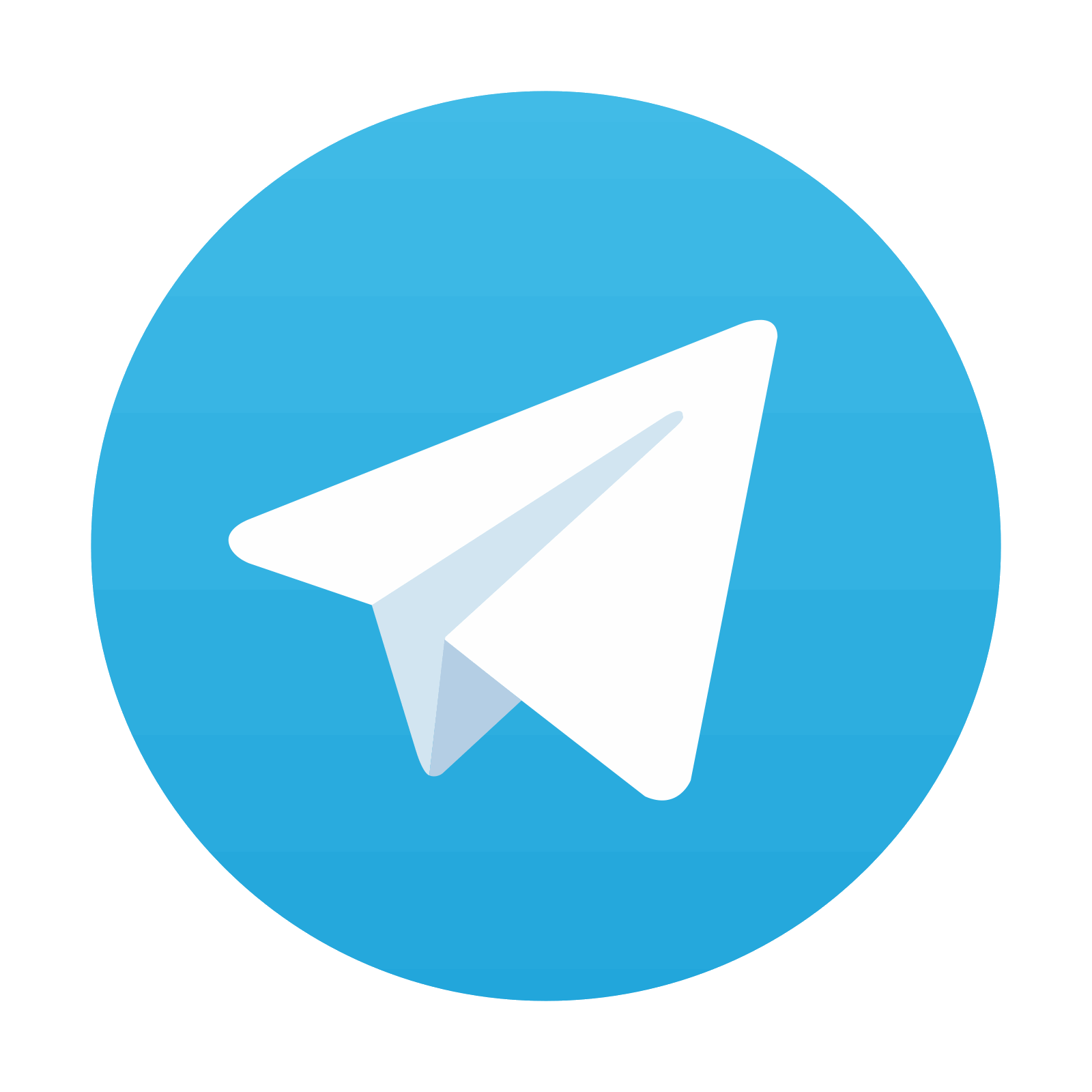
Stay updated, free articles. Join our Telegram channel
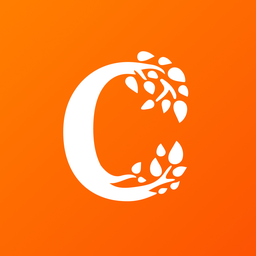
Full access? Get Clinical Tree
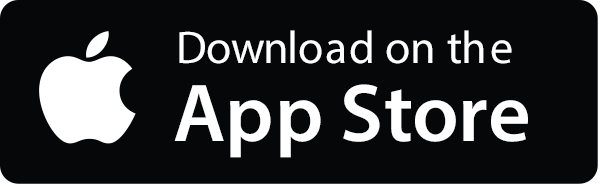
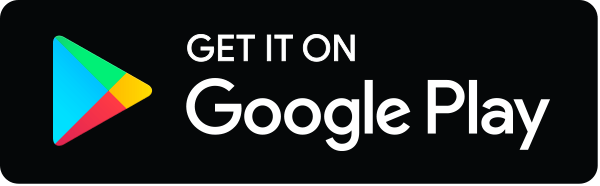