Tracer
First author, year
Type
Target/process
18F-FMISO
Rajendran, 2006 [14]
Nitroimidazole
Hypoxia
18F-FAZA
Mortensen, 2012 [15]
Nitroimidazole
Hypoxia
18F-HX4
Zegers, 2015 [16]
Nitroimidazole
Hypoxia
18F-EF5
Komar, 2014 [17]
Nitroimidazole
Hypoxia
62Cu-ATSM
Sato, 2014 [18]
Copper semicarbazone
Hypoxia
64Cu-ATSM
Grassi, 2014 [19]
Copper semicarbazone
Hypoxia
18F-FLT
Hoeben, 2013 [20]
Nucleoside
Proliferation, DNA synthesis
11C-4DST
Ito, 2015 [21]
Nucleoside
Proliferation, DNA synthesis
11C-MET
Wedman, 2009 [22]
Amino acid
Amino acid metabolism
18F-FAMT
Kim, 2015 [23]
Amino acid
Amino acid metabolism
18F-FET
Pauleit, 2006 [24]
Amino acid
Amino acid metabolism
18F-FMT
Burger, 2014 [25]
Amino acid
Amino acid metabolism
11C-Choline
Ito, 2010 [26]
Phospholipid precursor
Phospholipid biosynthesis
18F-FCH
Parashar, 2012 [27]
Phospholipid precursor
Phospholipid biosynthesis
15O-H2O
Komar, 2014 [17]
Water
Perfusion
68Ga-DOTATOC
Schartinger, 2013 [28]
Octreotide
Somatostatin receptor expression
18F-BPA
Tani, 2014 [29]
Boron-amino acid
Replicating cell/boron accumulation
18F-5-FU
Hino-Shishikura, 2013 [30]
Cytotoxic chemotherapy
Replicating cell/drug distribution
89Zr-Cetuximab
Heukelom, 2013 [31]
Antibody
EGFR expression, drug distribution
89Zr-U36
Börjesson, 2009 [32]
Antibody
CD44v6 expression
124I-F16SIP
Heuveling, 2013 [33]
Mini antibody
Fibronectin/angiogenesis
Hypoxia Imaging
Tumor hypoxia is associated with poor prognosis and resistance to treatment. Tumor hypoxia can be analyzed directly by measuring oxygen tension with an electrode, but this is an invasive procedure which does not take into account tumor heterogeneity. Hypoxia imaging on the other hand allows serial noninvasive assessment of tumor hypoxia, both of the primary tumor and of lymph node metastases. Multiple hypoxia PET tracers have been developed, mostly based on a nitroimidazole structure. These molecules freely diffuse through cell membranes but get trapped into cells in the presence of a low oxygen level [34, 35]. The most used hypoxia PET tracer is 18F-fluoromisonidazole (18F-FMISO) which has recently been reviewed by Rajendran and Krohn [36]. Several, generally small single center, 18F-FMISO PET studies have been published in HNSCC patients over the last 10 years (Table 5.2). In these studies, different parameters for quantification were used, but also different reference tissues, different treatment schedules, and different timing of follow up imaging. This complicates interpretation and hampers robust conclusions. However, several studies showed that patient with more hypoxic tumors had a worse outcome [14, 37, 41, 44, 46, 50]. Furthermore, early reoxygenation during chemoradiotherapy appears to be associated with a lower risk of recurrence [46, 50].
Table 5.2
18F-FMISO PET studies in HNSCC patients
Author | Year | Pt N | Treatment | Timing | Quantification parameters | Reference tissue | Prognostic value | Predictive value |
---|---|---|---|---|---|---|---|---|
Thorwarth [37] | 2005 | 15 | (C)RT | Baseline | TAC, TRP, perfusion, SUVmax, FHV | Blood | Yes | NA |
Rajendran [14] | 2006 | 73 | (C)RT / surgery ± PO(C)RT | Baseline | HV, TBRmax | Blood | Yes | NA |
Rischin [38] | 2006 | 45 | RT + tirapazamin vs. CRT | Baseline, week 4/5 | Qualitative > background | NR | No | Yes |
Eschmann [39] | 2007 | 14 | (C)RT | Baseline, 30 Gy | TAC, mean SUV, TMR | Muscle | NR | NA |
Nehmeh [40] | 2008 | 20 | NR | Baseline 2× | FHV | Blood | NR | NA |
Dirix [41] | 2009 | 15 | CRT | Baseline, week 4 | HV, TBRmax | Blood | Yes | NA |
Lee [42] | 2009 [43] | 20 | CRT | Baseline, week 4 | Qualitative > background | NR | No | NA |
Kikuchi [44] | 2011 | 17 | NAC followed by surgery or (C)RT | Baseline | SUVmax, TMR | Muscle | Yes | NA |
Yamane [45] | 2011 | 14 | NAC | Before + after NAC | SUVmax, TMR, HV | Muscle | NR | no |
Zips [46] | 2012 | 25 | CRT | Baseline week 1, 2, 5 | HV, baseline HF, SUVmax, TBRmax | Muscle | Yes | NA |
Bittner [43] | 2013 | 16 | CRT | Baseline, week 2 | HV | NR | NR | NA |
Henriques [47] | 2013 | 15 | RT | Baseline | TBRmax, FHV 3× | NR | NR | NA |
Okamoto [48] | 2013 | 11 | NR | Baseline 2× | SUVmax, TMR, TBR, HV | Muscle Blood | NR | NR |
Sato [49] | 2014 | 22 | NAC followed by surgery | Before surgery + before/during/after NAC | SUVmax | TMR | NR | Yes |
Wiedenmann [50] | 2015 | 16 | CRT | Before, week 2, 5 | TBRmax, HV | Blood | Yes | NA |
Where prognostic markers provide information about outcome of patients independent of treatment, predictive markers give information on the effect of a specific treatment strategy [51]. The prognostic value of hypoxia PET can potentially be used to guide treatment de-escalation in patients with nonhypoxic tumors with favorable prognosis and/or treatment escalation in patients with hypoxic tumors (Fig. 5.1). Currently a treatment de-escalation study is ongoing in patients with human papillomavirus (HPV) positive oropharynx cancers that are nonhypoxic at baseline or show early re-oxygenation on repeat imaging (ClinicalTrials.gov Identifier: NCT00606294). On the other end of the spectrum, in silico studies have demonstrated the feasibility of increasing radiotherapy dose to hypoxic tumor subvolumes [53–57]. Currently two randomized studies are comparing standard chemoradiotherapy with chemoradiotherapy using an increased radiation dose to hypoxic tumor subvolumes (ClinicalTrial.gov. Identifiers: NCT02352792 and NCT01212354).
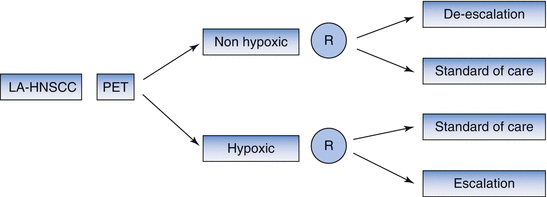
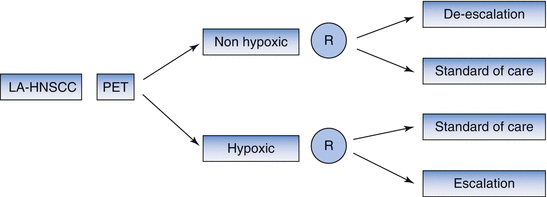
Fig. 5.1
Study design using hypoxia PET as prognostic marker. LA-HNSCC locally advanced head and neck squamous cell carcinoma, PET positron emission tomography, R randomization. Patients with LA-HNSCC undergo hypoxia PET imaging before start of treatment. Patients with nonhypoxic tumors are randomized between standard of care and an experimental treatment de-escalation regimen. Patients with hypoxic tumors are randomized between standard of care and an experimental treatment intensification regimen. Double enrichment design (After Freidlin et al. [52])
Several therapeutic strategies have been developed to reduce tumor hypoxia during radiotherapy, including carbogen and nicotinamide, tirapazamine, and nimorazol [58–60]. Hypoxia PET may have predictive value by identification of patients who benefit from hypoxia targeting treatment. This could be investigated by using a biomarker stratified study design (Fig. 5.2). Data from a sub study using 18F-FMISO PET suggested that patients with hypoxic tumors derived benefit from treatment with tirapazamin, a cytotoxic drug with selective toxicity towards hypoxic cells [38]. Currently an international randomized phase III trial comparing chemoradiotherapy plus nimorazol with chemoradiotherapy plus placebo in patients with locally advanced HNSCC uses a hypoxic gene signature as stratification factor but also tests predictive value of hypoxia PET in a subset of the patients (ClinicalTrials.gov Identifier: NCT01880359). However, nimorazole is a cheap drug with limited side effects, therefore it is doubtful if hypoxia PET is going to be implemented as predictive marker even if the positive and negative predictive values are high.
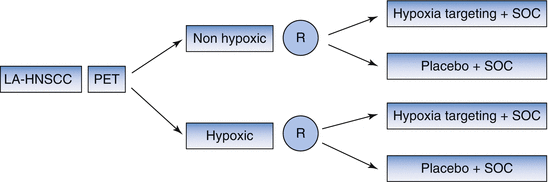
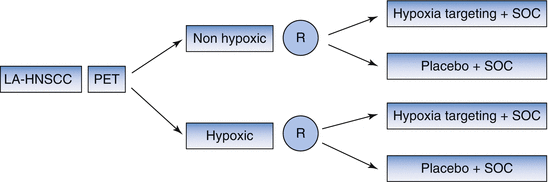
Fig. 5.2
Study design testing hypoxia PET as predictive marker. LA-HNSCC locally advanced head and neck squamous cell carcinoma, PET positron emission tomography, R randomization, SOC standard of care. Patients with LA-HNSCC undergo hypoxia PET imaging before start of treatment. Patients with nonhypoxic tumors as well as patients with hypoxic tumors are randomized between standard of care plus a hypoxia targeting drug and standard of care plus placebo. Biomarker stratified design (After Freidlin, et al. [52])
PET Imaging Using Radiolabeled Antibodies
PET imaging with radiolabeled monoclonal antibodies, also called immuno-PET, can potentially be used to select patient for targeted treatment and for drug development [5]. For HNSCC, the epidermal growth factor receptor (EGFR) blocking antibody cetuximab is the only targeted therapy shown to be effective, in combination with radiotherapy and in combination with chemotherapy [61, 62]. EGFR, also known as human epidermal growth factor receptor 1 (HER1), is a member of the human EGFR (HER) family that further consists of HER2, HER3, and HER4.
Preclinical research has shown that activation of HER3 after dimerization with HER2 limits activity of EGFR inhibition in HNSCC and that dual inhibition of EGFR and HER3 can overcome resistance to radiation and to EGFR inhibition [63, 64]. Several agents targeting HER3 are currently under investigation in clinical trials, including monoclonal antibodies directed against HER3, dual inhibitors of EGFR and HER3, and pan-HER monoclonal antibody mixtures and tyrosine kinase inhibitors. Immuno-PET using radiolabeled antibodies against EGFR and HER3 could be useful to provide information on availability of the drug target and distribution of therapeutic antibodies in HNSCC patients.
EGFR Imaging
EGFR expression determined by immunohistochemistry has prognostic value in HNSCC, but is not a predictive biomarker for efficacy of cetuximab [65]. This may be related to heterogeneity in EGFR expression but also to accessibility of the tumor to EGFR inhibitors. Tumor drug delivery is not solely dependent on expression of the target, but also determined by perfusion, permeability, interstitial pressure, and drug characteristics including size [66, 67]. Two preclinical studies investigating 64Cu-cetuximab PET imaging in xenograft models reported a correlation between tumor uptake of 64Cu-cetuximab and EGFR expression [68, 69]. A third study with 89Zr-cetuximab PET imaging in tumor bearing mice showed tracer uptake in EGFR positive tumors, but no correlation between tracer uptake and EGFR expression was found [70]. This may however be related to the tracer dose used [71].
Antibodies have a long half-life which implicates that in order to achieve a good tumor-to-background ratio and tumor-to-blood ratio, the optimal timing of imaging is around 7 days after tracer injection. To allow imaging within 24 h and repeat imaging early after start of treatment, antibody fragments of cetuximab (cetuximab-F(ab’)2) have been developed and radiolabeled for SPECT and PET imaging [72, 73]. Imaging studies in head and neck cancer xenograft models using 111In-cetuximab-F(ab’)2 SPECT have shown that localization of the tracer correlates with EGFR expression and that the model with the highest uptake was the most sensitive to cetuximab treatment [72, 74]. Furthermore, increased tumor tracer uptake was found after radiotherapy in a cetuximab sensitive HNSCC xenograft model, which was accompanied by translocation of EGFR to the tumor cell membrane [75]. On the other hand, in a cetuximab resistant tumor model, no increase in tumor tracer uptake after radiotherapy occurred. Finally, treatment of human HNSCC xenograft models with radiotherapy alone, cetuximab alone, or the combination demonstrated reduced tracer uptake in responding tumors while in resistant tumors an increase in tumor tracer uptake was found [75]. Therefore, translation of this molecular imaging technique to the clinic offers a promising tool for selecting patients who will benefit from treatment with cetuximab but it could also be useful as an early read-out of treatment efficacy. Three clinical studies have started using 89Zr-cetuximab PET imaging, one in HNSCC and two in colorectal cancer patients (ClinicalTrials.gov Identifiers: NCT01504815, NCT02117466, NCT01691391) [31, 76]. The head and neck cancer trial was initiated as a randomized phase II study comparing cisplatin with cetuximab and standard radiotherapy with redistributed radiotherapy in a two by two factorial design. One of the objectives was to evaluate the predictive value of 89Zr-cetuximab tumor uptake on a pretreatment PET scan [31]. Unfortunately the trial design has been changed and cetuximab treatment and cetuximab imaging are no longer part of the protocol (https://clinicaltrials.gov/archive/NCT01504815/2014_08_21/changes).
HER3 Imaging
The HER3 antibodies lumretuzumab and patritumab have been labeled for PET imaging [77, 78]. In a phase I study, 13 patients with solid tumors expressing HER3, determined by immunohistochemistry, underwent imaging with 89Zr-lumretuzumab PET before start of treatment with the same antibody [79]. Two patients with HNSCC were included in this study. The aim of the imaging part was to determine in vivo biodistribution and the ability of the antibody to target the tumor. In all patients, tracer uptake in tumor lesions was seen. Metastases in the bone and brain that were unknown were detected in three patients. Results of serial imaging during treatment to assess HER3 saturation are awaited. In another phase I study, dosimetry of 64Cu-DOTA-patritumab and receptor occupancy after a therapeutic dose patritumab were investigated [78]. Three out of six patients in the receptor occupancy cohort had a negative PET scan, likely because patients were not preselected for HER3 tumor expression. In the remaining patients, receptor occupancy was ~42 %. Larger studies are needed to assess predictive value of immuno-PET for efficacy of antibody therapy and/or for selecting the right treatment dose.
PET Imaging of Tumor Immunity
Cancer immunotherapy with immune checkpoint inhibitors has been a great breakthrough for melanoma, non–small cell lung cancer and other tumor types and has shown very promising early results in head and neck cancer [80, 81]. Antibodies directed at programmed death 1 (PD-1) and its ligand PD-L1 are currently investigated in phase III trials in HNSCC. To date there are no biomarkers that predict efficacy of immune checkpoint inhibition, although in some tumor types expression of PD-L1 using immunohistochemistry is associated with a higher response rate. Expression of relevant targets for immunotherapy may vary between and within tumor lesions, and over time. Molecular imaging offers a noninvasive platform for serial assessment of whole body target expression and antibody distribution. PET imaging of tumor immunity is in an early phase of development with no clinical data available yet. One imaging study is currently ongoing in patients with triple negative breast cancer, bladder cancer and non–small cell lung cancer using 89Zr-atezolizumab (PD-L1 antibody) PET before treatment with the same antibody (ClinicalTrials.gov Identifier: NTC02453984). Two preclinical studies already demonstrated feasibility and specificity of radiolabeled antibodies for PD-L1 imaging in tumor bearing mice [82, 83].
Another interesting strategy is to use molecular imaging as an early read-out of treatment response. PD1 and PD-L1 antibodies are supposed to act by augmenting the activity of tumor infiltrating cytotoxic T lymphocytes. Activated T lymphocytes express interleukin-2 (IL-2) receptor. SPECT imaging with radiolabeled IL-2 (99mTc- IL2) has successfully been used in patients for visualization of activated T lymphocytes in atherosclerotic plaques and in melanoma [84, 85]. For IL-2 PET imaging, which allows more sensitive and more precise quantification than SPECT, 18F-IL2 has been developed and validated preclinically [86, 87].
The subset of lymphocytes that can eliminate tumor cells are CD8 expressing cytotoxic T cells. Antibody fragments against murine CD8 have successfully been labeled with 64Cu and showed specific uptake in lymph nodes and spleen of antigen positive mice [88]. If this technique can successfully be translated to the clinic, it would allow to study in vivo tumor T cell infiltration which appears to be a prerequisite for immunotherapy to be effective.
Another important class of immune cells affecting tumor behavior are the tumor associated macrophages (TAMs). The subset of M2 macrophages appears to have a tumor promoting and cytotoxic T cell suppressive effect [89]. Macrophage depleting drugs are currently investigated in clinical trials. M2 macrophages specifically express the macrophage mannose receptor (MMR). Radiolabeled antibody fragments targeting MMR have been developed for PET imaging and showed specific uptake in tissues and tumors expressing MMR in mice [90]. MMR imaging could be helpful in the process of drug development, for patient selection, and as a read out for treatment efficacy.
Optical Imaging
Optical molecular imaging is a much more recent field of research, and evidence from clinical studies is still scarce. Optical imaging techniques use illumination with light of different wave lengths, ranging from safe ultraviolet range (350–400 nm), and the visible spectrum (400–750 nm) to infrared regions (750–1000 nm). Penetration of light is limited due to scattering and absorption, which vary substantially in different target tissues. Generally, in the range of 350–1000 nm, light penetration is deeper with increasing wavelength. Near-infrared (NIR) fluorescence imaging can visualize structures up to 8 mm below the surface, depending on the optical properties of the target tissue [91]. Several optical spectroscopy and imaging technologies have been and are currently investigated in HNSCC, including Raman spectroscopy, narrow band imaging, autofluorescence and exogenous fluorophore imaging, optical coherence tomography, confocal laser endomicroscopy, and confocal reflectance microscopy [92]. Optical imaging is currently investigated for its potential to differentiate malignant lesions from normal tissue, and from benign and premalignant lesions. Optical imaging can be used during endoscopy but also intraoperatively to guide surgical resection margins. However, in cancer diagnosis, most optical techniques are either difficult to apply in vivo (i.e., Raman spectroscopy) or have not shown to yield sufficient specificity to support routine clinical use. Only in certain fields optical imaging techniques have shown to aid the clinician in diagnostic procedures. For instance, narrow band imaging helps to identify (pre)malignant lesions in head and neck cancer [93]. Here we present examples of molecular optical imaging using fluorescently labeled molecules that target specific tumor characteristics to improve the contrast between cancer and non-cancer tissue.
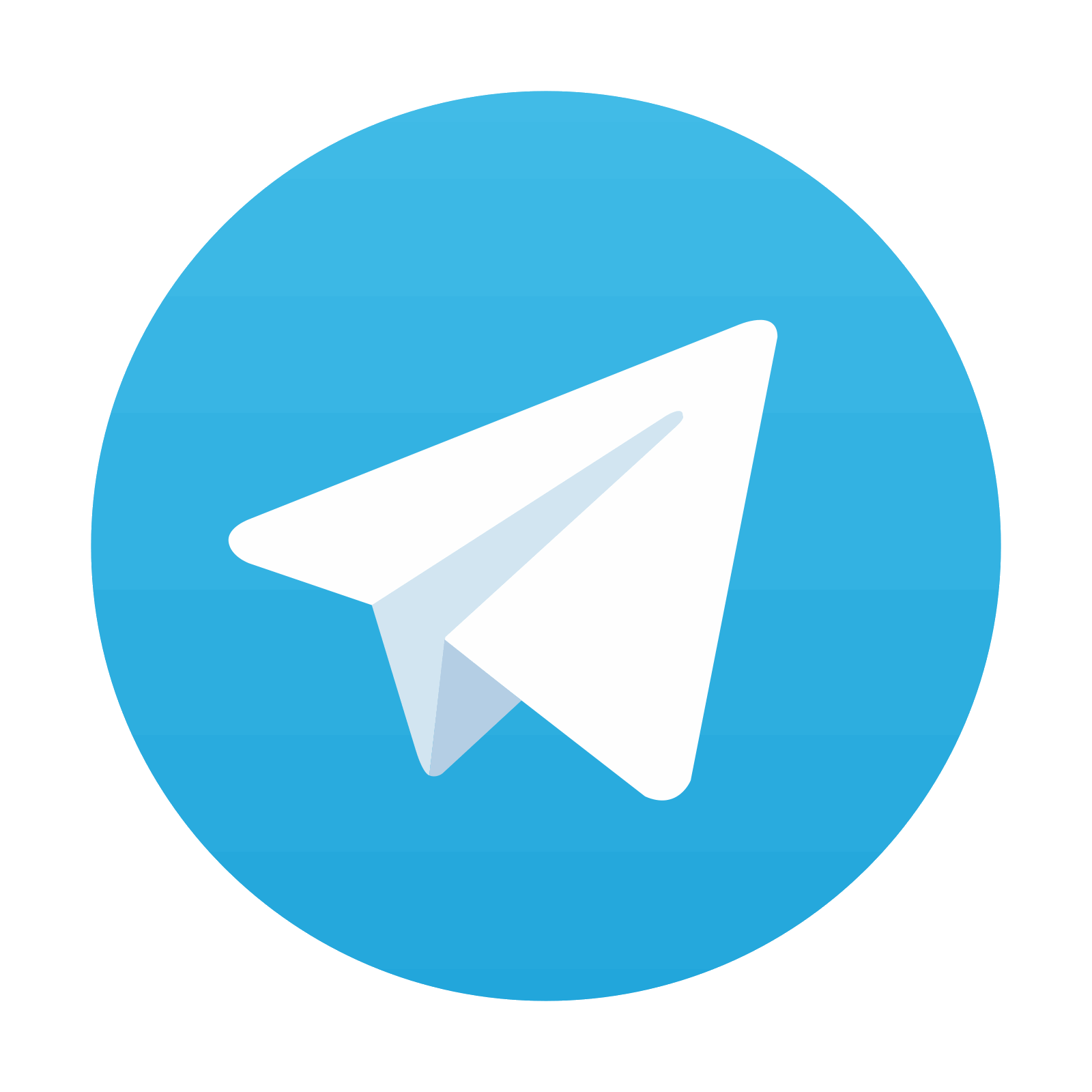
Stay updated, free articles. Join our Telegram channel
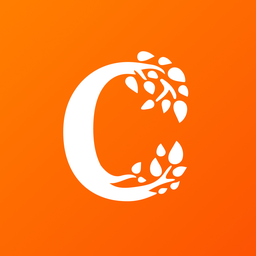
Full access? Get Clinical Tree
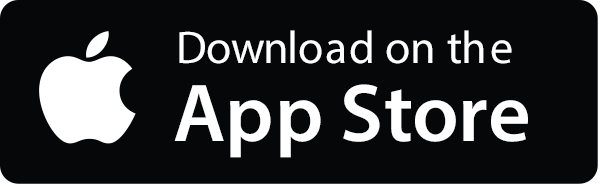
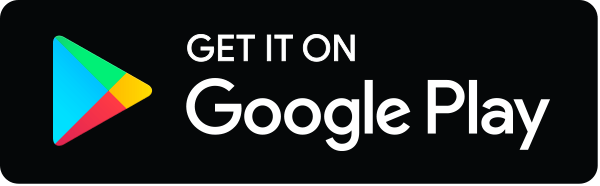