Tumor
Genes
Frequency
Citations
PTC
BRAF
40–50%
RET/PTC
10–20%
RAS
10–20%
TRK
5–13%
[16]
TERT
9%
[2]
EIF1AX
2%
[2]
FTC
RAS
20–50%
PAX8/PPARG
30–40%
[19]
P13K/AKT
6%
PTEN
7%
[20]
IDH1
5%
[22]
TERT
17.1%
BRAF
Genetics
BRAF mutations are found in a wide variety of tumors including 40–70% of melanomas, 10% of colorectal tumors, as well as ovarian, breast, and lung tumors [25, 26]. BRAF germline mutations have been identified in Leopard syndrome, a developmental disorder with multiple granular cell tumors, pulmonary stenosis, deafness, and cardiac conduction abnormalities [27, 28]. In thyroid cancer, BRAF mutations are limited to papillary, poorly differentiated, and anaplastic carcinomas. There is no evidence of involvement of BRAF in follicular thyroid carcinoma (FTC) or benign thyroid nodules [29].
The members of the RAF family proteins are cytoplasmic serine/threonine protein kinases in the mitogen-activated protein kinase (MAPK) signaling cascade. The cascade begins with RAS, a small membrane-bound G protein, activating RAF to the plasma membrane. The activated RAF phosphorylates MAP-ERK kinase (MEK), which in turn phosphorylates and activates extracellular signal regulated kinases (ERK). ERK has over 150 nuclear and cytosolic targets, which include transcription factors that regulate cellular differentiation, proliferation, senescence, and survival [30]. There are three isoforms of RAF: ARAF, BRAF, and CRAF (RAF-1). BRAF, v-raf murine sarcoma viral oncogene homolog BI, is the most frequently mutated in the kinase superfamily and is the strongest MAPK activator [28, 31–33]. The majority of BRAF mutations in thyroid carcinomas are at V600E (95%) [34]. Wild-type BRAF has a hydrophobic interaction with residues G465 to V472 located in the ATP-binding site, which keeps the kinase activity inactivated until appropriately signaled by RAS. BRAF V600E is created by an oncogenic T to A transversion at nucleotide 1799, which changes glutamate to valine at codon 600 of the kinase activation segment [26, 35, 36]. This mutation causes the BRAF kinase to fold into the catalytically active formation, which increases its activity almost 500-fold [37]. Other rare BRAF activating mutations associated with differentiated thyroid carcinomas includes small in-frame insertions and deletions that make up a small minority of the BRAF mutations (1–2%) [38]. Point mutations around codon 600 include a K601E mutation found primarily in the follicular variant of PTC (FVPTC) [39, 40]. AKAP9–BRAF is a paracentrically inverted oncogenic rearrangement found on chromosome 7, which is associated with PTCs arising in the setting of radiation exposure [41].
Clinical and Pathology Characteristics
BRAF is the predominant genetic mutation in adult sporadic PTC, making up 29–69% of cases. The majority of studies from the United States report the frequency of BRAF mutations in PTCs between 40 and 50% [4–8]. BRAF mutant tumors have well developed nuclear features typically associated with PTC. BRAF mutant tumors have a high degree of lymphocytic infiltration and are less frequently associated with psammoma bodies (20%) than tumors with RET/PTC rearrangements [42]. Tumors with BRAF mutations are associated with aggressive histologic features such as tall cell variant, older patient age, lymph node metastasis, and extrathyroidal extension [2, 43, 44]. Tall cell variant PTC, an aggressive subset of PTC, has been found to have a higher frequency of BRAF mutations (70–80%) [45–47]. In a study of 190 fine needle aspiration specimens, 73 of which were positive for BRAF mutations, Xing et al. reported BRAF mutations predicted extrathyroidal extension (23%, n = 17) versus wild type (11%, n = 13), thyroid capsular invasion (29%, n = 21 vs. 16%, n = 19), and persistence or recurrence of disease (36%, n = 19 vs. 12%, n = 9) [48]. In a recent landmark integrated genomic study of 496 PTCs from The Cancer Genome Atlas (TCGA), two major subgroups of PTCs were identified, one of which is driven by BRAF and associated with tall cell variant tumors [2]. However, it is important to note that BRAF mutant tumors are complex and not a homogenous group. Thus additional alterations occurring within the context of BRAF mutations will need to be considered for tumor behavior and prognosis [2].
BRAF mutant tumors are also associated with I131 resistance [49]. This resistance is likely due to silencing of thyroid-specific iodine expressing genes, such as sodium iodine symporter, apical iodide transporter, thyroperoxidase, and thyroglobulin [50, 51]. In elegant preclinical studies, inhibition of BRAF and the downstream signaling cascade resulted in cells regaining their sensitivity to I131 [52, 53]. I131 response was further enhanced by dual inhibition of the pathway using a BRAF and MEK inhibitor [51]. Further clinical studies will be needed to determine if this dual inhibitor approach may improve sensitivity to I131 in patients as monotherapy did not have a significant effect on patient outcome [52, 53].
RET/PTC
Genetics
The RET (rearranged during transfection) proto-oncogene is a single-pass transmembrane tyrosine kinase located on chromosome 10q11.2 [54, 55]. Activation of RET is highly regulated by ligand-binding dimerization with glial-derived neurotrophic factor family ligands [56]. This proto-oncogene is integral in regulating cell growth and survival [55]. It is essential in the development of the sympathetic, parasympathetic, and enteric nervous systems, the gastrointestinal lymphoid system, as well as the kidneys and testis. Germline loss of function RET mutations result in impaired formation of the enteric nervous system and congenital agangliosis of the colon (Hirschsprung’s disease) [57]. Wild-type RET is necessary for the development of neuroectodermal cells that become thyroid C cells [58].
RET is activated when its 3′ tyrosine kinase intracellular domain fuses with the 5′ end of an alternate gene with a coiled-coil domain. This fusion creates ligand-independent dimerization. The new chimera does not contain the intracellular juxtamembrane domain, which is an important component of the auto-inhibited RET dimer interface [59, 60]. The RET/PTC rearrangement contains intrinsic and constitutive tyrosine kinase activity, which results in subcellular re-localization where cell growth and survival are constitutively activated [61–63].
There are at least 15 RET/PTC rearrangements identified in PTC [56, 64]. RET/PTC1 rearrangements are the most common (60–70%), followed by RET/PTC3 (20–30%), while the remaining rearrangements make up less than 5% of all RET/PTC rearrangements [65]. The tumorigenicity of the RET/PTC rearrangements may be attributed to the RET fusion partners, such as PRKAR1A. PRKAR1A is a cyclic AMP-dependent protein kinase type I regulatory subunit, a tumor suppressor gene mutated in Carney complex and CCDC6 (coiled-coil domain-containing protein 6) [66, 67]. RET/PTC1 is a balanced intrachromosomal inversion with CCDC6, formally known as H4/D10S170, on chromosome 10 [61, 65, 68, 69]. Loss of an allele of CCDC6 as a result of this rearrangement is believed to promote transcription and thyroid hyperplasia [70]. RET/PTC2 is the result of an interchromosomal translocation with PRKAR1A [71]. RET/PTC3 is an intrachromosomal inversion with nuclear receptor coactivator gene-4 (NCOA4), formally known as ELE1/ARA70/RFG, a transcriptional coactivator on chromosome 10 [10, 64]. RET/PTC2 and less frequent RET rearrangements are interchromosomal translocations [72]. RET/PTC rearrangements can be clonal rearrangements, affecting all cells of a tumor or non-clonal, that is specific to a single or small fraction of cells within a tumor.
Clinical and Pathology Characteristics
Overall, RET/PTC rearrangements are more likely associated with classic papillary thyroid architecture and microadenomas [42]. Psammoma bodies, lamellated calcified collections of necrotic papillae, have been noted in 100% of RET/PTC rearrangement associated PTC compared to only 20% of BRAF mutant PTC [42, 73]. RET/PTC1 rearrangements are more common in papillary microcarcinomas seen in younger patients with PTC and when present are associated with a higher frequency of lymph node metastasis and classic histology. The RET/PTC3 rearrangement is most commonly seen in solid variant PTC [74–76]. Increased incidence of RET/PTC rearrangements (50–80%), especially RET/PTC3, are seen in patients with a previous history of radiation [9, 71, 77–81]. RET, CCDC6, NCOA4 genes are all adjacent on chromosome 10. Ionizing radiation promotes double-strand DNA breaks, catalyzing a nonhomologous recombination between these juxtaposed genes [82, 83]. These findings have been corroborated in patients after exposure to the nuclear accident at Chernobyl, atomic bombings at Hiroshima and Nagasaki, Semipalatinsk nuclear testing site, and children who have been irradiated for benign conditions [84]. The incidence of RET/PTC rearrangements is especially increased in children, likely due to rapid thyroid cell proliferation.
RAS
Genetics
Members of the rat sarcoma viral oncogene homologue (RAS) superfamily of GTP-binding proteins include three genes: HRAS (chromosome 11p15.5), KRAS (chromosome 1p13.2), and NRAS (chromosome 12p12.1) [85]. These genes, which are nearly ubiquitously expressed and conserved across species, encode membrane-associated guanine nucleotide-binding proteins (p21ras). RAS, an oncogene, encodes a small GTPase that cycles between an active (GTP-bound) and inactive (GDP-bound) state. Activated RAS then stimulates a diverse cascade of downstream effectors and signaling networks [86]. These proteins regulate extracellular receptor signals to influence downstream intracellular pathways, including RAF/MAPK, PI3K/AKT, and others. RAS is integral in the modulation of cellular differentiation, survival adhesion, migration, apoptosis, and senescence [36, 87, 88].
RAS mutations are the second to BRAF for the most common genetic mutation in thyroid cancers. RAS mutations have been credited with the dramatic increase in thyroid carcinomas over the last 40 years [89]. Mutations in RAS associated with differentiated thyroid carcinoma most commonly occur in codons 12, 13, and 61 (99%). Rarely, other mutations such as amplifications and polymorphisms may occur [90]. Codons 12 and 13 code for the GTP-binding domains, and codon 61 encodes the GTPase domain; therefore, mutations in these codons dampen the GTPase activity. As a result, these mutations disrupt the RAS and guanine nucleotide interaction which results in constitutively active RAS [91]. KRAS mutations are most commonly found in codon 12 (65%) and 95% of NRAS mutations are in codon 61. Codon 61 mutations in NRAS (chromosome 1p13) and HRAS (chromosome 11p11), which inactivate the autocatalytic GTPase, are the most common in thyroid cancers [38]. This inactivation results in constitutive RAS activation and chronic stimulation of MAPK, PI3K/AKT, and other downstream signaling pathways. NRAS (8.5%) is the predominant mutation in PTCs, with HRAS (3.5%) and KRAS (1%) composing a smaller proportion of these tumors [2].
Clinical and Pathology Characteristics
RAS mutations are seen in 10–20% of PTCs [11–15]. The recent Cancer Genome Atlas analysis divided PTCs into BRAF-like, discussed previously, and RAS-like subgroups. As expected, RAS-like tumors were significantly associated with RAS mutations but more importantly showed concurrent activation of PI3K/AKT and MAPK signaling. RAS-like tumors were associated with follicular variant (FV) PTC and lower risk of recurrence [2, 92, 93]. Psammoma bodies, extrathyroidal extension, and lymph node metastasis are rarely seen with RAS mutations. RAS mutant PTCs are more frequently encapsulated, with a female preponderance and tendency toward increased size [42]. RAS mutations make up 20–50% of conventional-type FTCs and 20–40% of conventional-type follicular adenomas, which will be discussed in more depth later in this chapter [94–96]. Even though FVPTCs are genotypically and histologically more similar to FTCs rather than classic PTC, they are currently still classified with PTCs [97, 98]. Multiple studies have correlated RAS mutations with increased bone metastasis, aggressiveness, and mortality [15, 99, 100]. However, RAS mutations are seen in a significant number of benign follicular adenomas and low risk FVPTC [91, 101–103]. The impact on clinical prognosis and pathological classification of thyroid cancers with a RAS mutation continues to be uncertain, and further evaluation into this molecular subgroup is warranted.
TRK
Genetics
Neutrotrophic receptor-tyrosine kinase 1 (NRTK1, TrkA) is a proto-oncogene located on chromosome 1q21–22. This gene encodes for a transmembrane tyrosine kinase receptor with high affinity for nerve growth factor (NGF). Its wild type expression is important for development of the central and peripheral nervous system, especially for the neurons of sensory spinal and cranial ganglia of neural crest origin [104, 105]. In addition, it promotes proliferation of lymphocytes, keratinocytes, prostate cells, and others cell types [106–108].
Binding of the TRTK1 receptor by NGF results in dimerization and autophosphorylation of five tyrosine residues. Autophosphorylation results in elevated NTRK1 activity and activation of downstream signaling cascades, including RAS, PI3K, phospholipase C-gamma, and MAPK [109]. Rearrangements of NRTK1 result in a fusion of the tyrosine kinase domain to the amino terminus of different activating genes which results in aberrant expression of chimeric oncogenes. The tyrosine kinase domain becomes constitutively active, leading to deregulation of cell proliferation and oncogenic transformation [110]. The most common fusion partner forming chimeric oncogenes with NRTK is TPM3 (isoform of non-muscle tropomyosin) on chromosome 1q31 creating the TRK (thyroid oncogene receptor kinase) oncogene [110–112]. Less frequently involved is TPR (translated promotor region), which encodes a large protein of a nuclear pore complex involved in mRNA export on chromosome 1q25. When mutated, the TPR gene product forms the oncogene TRK-T1 and TRK-T2. Lastly fusion products involving TFG (TRK-fused gene) on chromosome 3q12 forms the TRK-T3 oncogene [113–119]. These fusion interactions result in proteins folded into alpha helices, which are further wound into a super helix that promote protein dimerization and constitutive activation [120]. No cytogenetic studies have been performed on NTRK1 rearrangements in PTC [120]. However, mutations are likely to be due to intrachromosomal inversion, preferentially with chromosome 1 in NTRK1 [121].
Clinical and Pathology Features
TERT
Genetics
Telomerase activation is a hallmark of cancer in up to 80% of malignant tumors [123–125]. Telomerase, a RNA-dependent DNA polymerase, contributes to cell immortality by lengthening the telomeres at the ends of chromosomes and decreasing apoptosis by protecting important chromosomal DNA [23]. Telomerase reverse transcriptase (TERT) is the rate-limiting step in transcriptional regulation of the telomerase complex. Oncogenic transformation in cells with abnormal telomerase results most often from deregulation of TERT transcription, resulting in TERT overexpression [126]. Mutations in the promotor region enhance TERT activity [124, 125]. Frequent mutations have been found in TERT promoter regions in melanomas, gliomas, bladder, and thyroid cancers [127–129]. Normal thyroid tissue and benign lesions do not express telomerase, and reactivation of this gene may play an important role in thyroid carcinoma [24, 127, 130, 131]. This mutation is reported to be present in 7.5–12% PTC and 17.1% FTCs [23, 24].
Two focuses for mutations have been identified at 124 and 146 base pairs upstream from the ATG start codon, named C228 and C250, respectively. C228T, the most common mutation (87%), represents a C to T nucleotide change [23]. Less commonly identified are C228A (C to A conversion) and C250T (C to T conversion) substitutions [2]. These point mutations create a consensus binding motifs site for E-twenty-six (ETS)/ternary complex transcription factors, which can also be upregulated by the MAPK pathway [23].
Clinical and Pathology Characteristics
Tumors with TERT promoter mutations are associated with an increase in aggressiveness of the tumor and are seen in conjunction with a high frequency of BRAF and RAS mutations up to 60% [24, 127, 130, 131]. There is an observed association with older patient age, larger tumor size, more distant metastasis, and higher stage. Although TERT promoter mutations were found in less-differentiated PTCs, there has been no identified correlation between TERT mutations and vascular invasion, extrathyroidal extension, or lymph node metastasis [2]. The presence of this mutation did have an increase in disease-specific mortality [24]. At follow up, Melo et al. showed TERT promoter mutations to be more likely to have persistent disease, as well as correlate with male gender and lymph node metastasis [24].
Other Significantly Mutated Genes
Recent whole exome sequencing of 402 tumor and normal pairs of PTC samples has provided unprecedented insight into the landscape of PTCs and the opportunity to evaluate less common somatic mutations [2]. Eukaryotic translation initiation factor 1A, X-linked (EIF1AX), was found to be mutated in six PTC tumors that lacked other known drivers, suggesting that EIF1AX may be a novel cancer gene in PTC [2]. Additionally genes involved in DNA repair, including PPM1D (protein phosphatase, Mg2+/Mn2+ dependent 1D) and CHEK2 (checkpoint kinase 2), were associated with higher mutation density unadjusted for age and high-risk patients [2]. Findings of additional DNA repair-associated genes may suggest that defects in DNA repair may be associated with more aggressive PTCs.
Follicular Thyroid Carcinoma
Follicular thyroid carcinoma (FTC) makes up 20% of differentiated thyroid cancers. The leading genetic alterations in FTC are RAS, PAX8/PPARG, IDH1, and the PTEN/PI3K/AKT pathway.
RAS
Genetics
The predominant mutation in the majority of reports of RAS mutations in FTC are NRAS, with lesser contributions from HRAS and KRAS [11, 18, 103, 132]. The most common NRAS point mutation includes a mutation changing glutamine to arginine (CAA → CGA; Q61R), followed by a mutation converting glutamine to lysine (CAA → AAA; Q61K) [91, 92, 133]. As mentioned above, RAS mutations are present in 20–40% follicular adenomas and 30–45% of FVPTC cases. In addition, 20–40% of poorly differentiated carcinomas and 20–30% of anaplastic carcinomas harbor RAS mutations [17, 134]. Explanations for RAS mutations throughout every stage of thyroid nodule include RAS as a de-differentiation factor from well-differentiated thyroid carcinomas versus RAS-positive follicular adenomas being precursor lesions to thyroid carcinoma [100, 135, 136].
PAX8/PPARγ
Genetics
PAX8/PPARγ is the result of a translocation between chromosome 2 and 3, resulting in t(2;3)(q13;p25). This translocation results in an in-frame fusion of a thyroid-specific paired box domain transcription factor (2q13) and PPARγ’s translated reading frame, which leads to the overexpression of fusion proteins [137, 138]. Expression of the PAX8/PPARγ fusion protein (PPFP) is controlled by PAX8’s upstream fusion protein. Expression of PPFP correlates with the tumor differentiation, high expression in well-differentiated tumors, and low expression in poorly differentiated tumors [137]. PAX8, paired box 8 gene, is integral to thyrocyte development. PAX8 controls expression of the sodium iodide symporter, thyroglobulin, and the TSH receptor. Therefore, PAX8 is an important regulator of thyroid differentiation, development, and growth [139, 140]. During human development, PAX8 is expressed in thyroid, kidney, and neural tissues; but in adulthood, expression is limited to thyroid tissue [141, 142].
PPAR (peroxisome proliferator-activated receptor) is a subfamily of the steroid/thyroid hormone nuclear receptor superfamily. Its role is to regulate adipogenesis and insulin sensitization via multiple cell signaling pathways [143, 144]. PPARγ is one of four subtypes of PPAR, the others include α, β, and δ. PPARγ modulates transcription by recruiting coactivators to DNA binding sites to increase transcription and interacts with corepressors to result in gene silencing. PPARγ is activated by thiazolidinediones, a class of medications used to treat diabetes [145]. Another PPARγ translocation associated with differentiated thyroid carcinoma is CREB3L2-PPARγ, resulting from a t(3;7)(p25;q34) chromosomal rearrangement of the transactivation domain of the CREB3L2 gene and PPARγ [146]. Multiple other genes have been identified that translocate with PPARγ at chromosome 3p25 [147].
Clinical and Pathology Characteristics
PAX8/PPARγ gene rearrangements comprise 30–40% of conventional-type FTCs. This translocation has been detected in follicular adenomas, which decreases the utility for using this rearrangement to diagnose FTC [19]. FTCs with PAX8/PPARγ tend to present at a younger age with smaller tumors, solid or nested growth pattern, and frequent vascular invasion [19, 40, 101, 148].
PI3K/AKT
Genetics
The PI3K/AKT cellular pathway is involved in regulation of glucose uptake, metabolism, growth, cellular motility, and crucial cellular functions. Phosphoinositide-3 (OH) kinase (PI3K) phosphorylates the beta-hydroxyl group of phosphatidylinositol inositides generating phosphatidylinositol-3, 4, 5-trisphosphate (PIP3). When activated by PIP3, phosphoinositide-dependent kinase 1 (PDK1) phosphorylates and activates AKT, a serine/threonine protein kinase also known as protein kinase B (PKB), mobilizing PDK1 to the cell membrane [149, 150]. Activated AKT then phosphorylates downstream effectors to promote cell proliferation and survival [20]. Phosphate and tensin homologue (PTEN) protein terminates this signaling cascade by degrading PIP3 via dephosphorylation [151].
PI3K is composed of a regulatory subunit (p85α, p85β, and p55γ) and a p110 catalytic subunit (α, β, γ, and δ). Thus far, only carcinoma-producing mutations have been identified in the p110α catalytic subunit [149]. PIK3CA mutations in FTC are relatively infrequent, occurring in only 6% of FTCs [20, 21]. The identified mutations (E542K, E545K, and H1047R) are in the helical (exon 9) and kinase (exon 20) domains [20, 152]. More commonly in FTC, PIK3CA and PIK3CB are amplified rather than mutated [21]. Approximately 25–45% of FTCs contain these amplifications as defined by a copy number gain of four or more [20, 21]. This amplification is due to duplication of the gene or chromosome 3 region, rather than aneuploidy. PIK3CA and PIK3CB result in an increase of AKT phosphorylation [152]. These findings are also prevalent in anaplastic thyroid carcinoma [20, 21].
PTEN
PTEN is a dual specificity phosphatase and tumor suppressor gene that negatively regulates the PI3K/AKT signaling pathway via dephosphorylation of the three positions on phosphoinositides [149]. Germline mutations of PTEN on chromosome 10q-22q24 result in Cowden’s syndrome, an autosomal dominant disorder. Mucocutaneous manifestations of Cowden’s syndrome include multiple hamartomas, specifically trichilemmomas, mucocutaneous papillomatous papules, as well as acral and palmoplantar keratosis. At least 66% of those affected with the disorder have breast or thyroid cancers, specifically FTCs [153]. Sporadic PTEN mutations occur at approximately 7% of FTCs [20]. More commonly found is methylation of the PTEN promoter that results in silencing of the gene [149]. In addition, loss of heterozygosity has been identified in up to 26% of FTCs, with homozygous deletions being rare [154–156]. PTEN silencing results in constitutive activation of the PI3K/AKT pathway.
IDH1
Isocitrate dehydrogenase 1 (IDH1) is a key enzyme in the tricarbocyclic acid cycle (TCA or Krebs cycle), which catalyzes the oxidative decarboxylation of isocitrate to produce alpha-ketoglutarate [157]. IDH1 is found within the cytoplasm, whereas IDH2 is located in the mitochondria [36]. IDH1 mutations associated with FTC occur in highly conserved residues and include G70D, I130M, H133Q, V178I, and V71I [22, 158]. G70D, I130M, and H133Q are located near the substrate-binding site, R132. The wild type codon R132 contains a highly conserved arginine integral to the catalytic activity of IDH1. The R132 residue is the location where the isocitrate’s α- and β-carboxylate binds in order to produce α-ketoglutarate. Mutations in this region likely result in decreased catalytic activity of the enzyme. The significance of the V178I mutation is still unclear [158]. There have been no associations found between the presence of IDH1 mutations and clinical features such as age, gender, tumor size, extrathyroidal invasion, and presence of metastases [158]. IDH1 mutations have been identified in a small number of papillary and follicular-type PTCs, as well as anaplastic and Hürthle cell carcinomas [36].
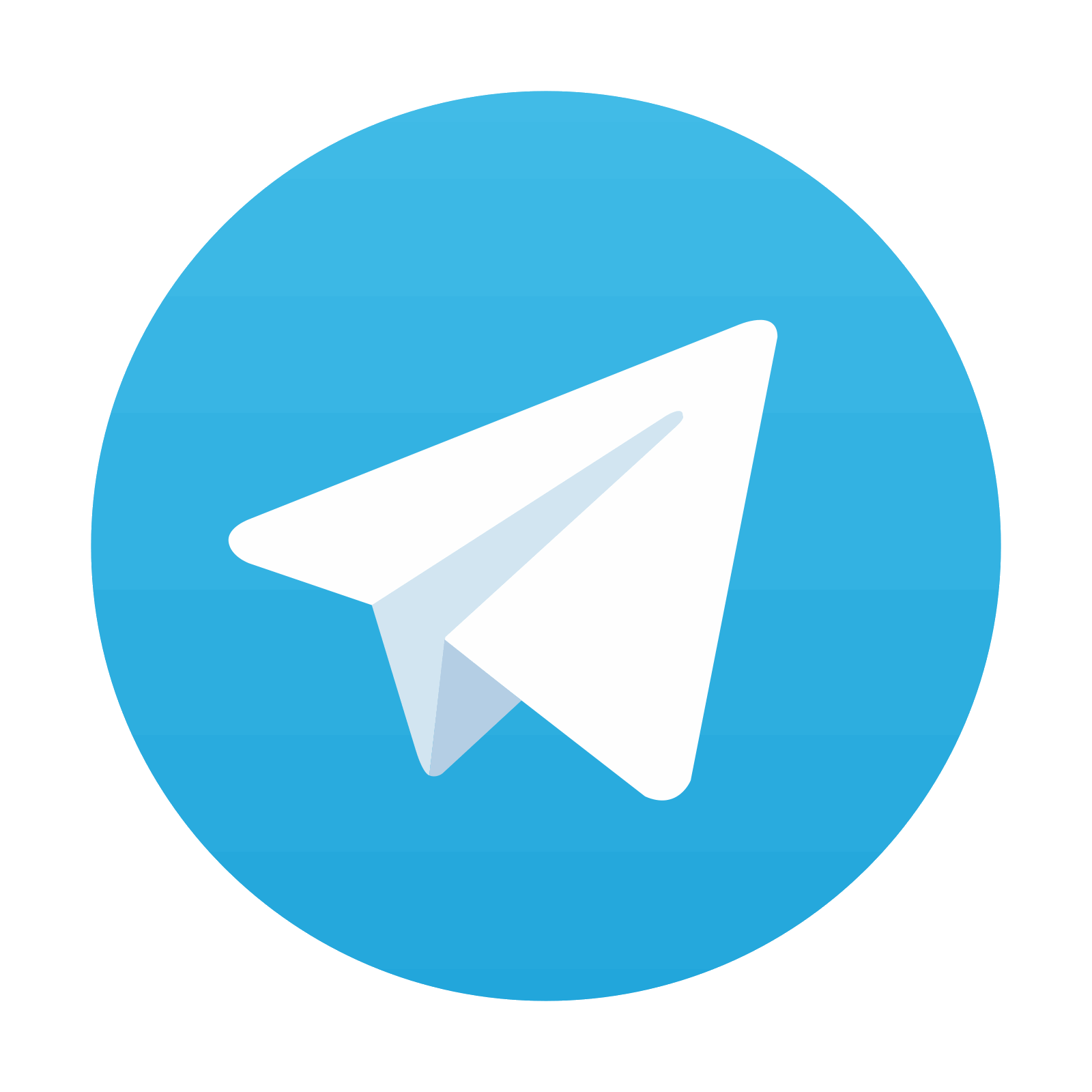
Stay updated, free articles. Join our Telegram channel
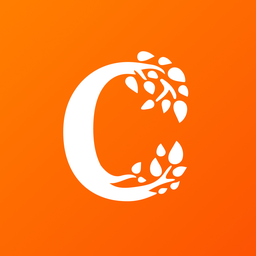
Full access? Get Clinical Tree
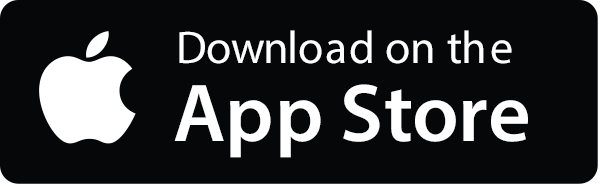
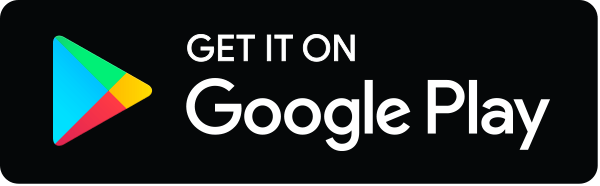