


LEUKEMIC STEM CELL
An important emerging concept in the pathobiology of leukemia is the existence of a leukemic stem cell. In normal hematopoietic development, there is a rare population of hematopoietic stem cells that have self-renewal capacity and that give rise to multipotent hematopoietic progenitors. These multipotent myeloid or lymphoid progenitors do not have a self-renewal capacity but mature into normal terminally differentiated cells in the peripheral blood. It is hypothesized that there is a leukemic stem cell that has limitless self-renewal capacity and that gives rise to clonogenic leukemic progenitors that do not have self-renewal capacity but are incapable of normal hematopoietic differentiation. Hypothesized functional differences between the rare leukemic stem cells and the bulk of derived leukemic progeny such as increased quiescence or engagement within a protective niche are believed to provide an intrinsic chemoresistance.
The first convincing evidence in support of the existence of a leukemic stem cell was derived from experiments in which human leukemic cells were injected into immunodeficient nonobese diabetic mice with severe combined immunodeficiency disease (NOD-SCID) mice.1,2 These data show that the resultant leukemias are derived from as few as 1:1000 to 1:10,000 cells, indicating that there is a rare population of human leukemic cells that have self-renewal capacity in this assay. These cells have similar immunophenotypes to normal self-renewing hematopoietic progenitors and suggest that the leukemogenic mutation occurs in a hematopoietic stem cell. In support of this hypothesis, clonal cytogenetic abnormalities, such as the t(9;22), have been detected in primitive hematopoietic progenitors such as CD34+CD38– cells.3
However, this paradigm has been recently challenged and revised. First, new protocols that enhance engraftment of human leukemia in the xenotransplant setting have been described, suggesting the importance of homing and the role of the microenvironment.4 Data also show that it may be the leukemic oncogenes themselves that confer properties of self-renewal. In a murine system, transduction of the leukemia oncogenes mixed-lineage leukemia 1/eleven nineteen leukemia (MLL/ENL), monocytic leukemia zinc finger protein/transcriptional intermediary factor 2 (MOZ/TIF2) or MLL/ALL1 fused gene from chromosome 9 protein (AF9) can confer properties of self-renewal to purified committed hematopoietic progenitors that have no capacity for self-renewal.5,6 HOX family and NOTCH genes, frequent mutational targets in acute leukemia as discussed later in this chapter, have been found to have significant roles in hematopoietic stem cell self-renewal.7 Secondary mutations may also enable activation of similar self-renewal pathways. For example, an analysis of cells from acute myeloid leukemia (AML) blast crisis in chronic myeloid leukemia (CML) shows a shift in the leukemic stem cell to an immunophenotype of a committed myeloid progenitor and concurrent nuclear localization of β-catenin, a process thought to increase stem cell self-renewal.8 One of the major goals is the identification of transcriptional programs, genes, and pathways that confer limitless self-renewal and may be targets for therapeutic intervention.
ELUCIDATION OF GENETIC EVENTS IN ACUTE LEUKEMIA
The search for causative mutations in acute leukemia has accelerated in recent years due to the availability of new means for evaluating genome integrity in leukemic cells. The bulk of known translocations and deletions were found by analyzing conventionally stained chromosomal banding patterns of karyotypes. The classical karyotypic analysis is able to identify lesions with a resolution of 5 to 10 Mb. These include balanced reciprocal chromosomal translocations, such as t(8;21)(q22;q22) or t(15;17)(q22;q21); internal deletions of single chromosomes, such as 5q- or 7q-; gain or loss of whole chromosomes (+8 or −7); or chromosome inversions, such as inv(3), inv(16), or inv(8). Array-based technologies such as comparative genomic hybridization (CGH) and single-nucleotide polymorphism (SNP) arrays allow detailed (<35 kb) mapping of unbalanced insertions or deletions.9,10 Array CGH determines DNA copy gain or loss by comparing the hybridization of sample DNA to a series of clones or oligonucleotides from regions throughout the genome bound to a chip with a normal reference DNA sample. SNP arrays differ in that oligomers of SNP sequences representing known alleles throughout the genome are used in the array to measure changes in expected genotype and copy number. Finally, low-cost high-throughput sequencing and microarray-based resequencing techniques have allowed for the identification of new somatic mutations at the single-nucleotide level and are making enormous inroads into the pathogenesis, prognosis, and classification of leukemias with normal cytogenetics. Worldwide initiatives to sequence large numbers of cancer genomes, including leukemia subtypes, are being coordinated and cataloged through the International Cancer Genome Consortium (http://www.icgc.org) and the Cancer Genome Atlas (TCGA) (https://tcga-data.nci.nih.gov/tcga).12
Although intensive effort has focused on chromosomal translocations in leukemia, in part because of their high frequency in various kinds of leukemia, it has become increasingly clear that point mutations play an important role in a spectrum of leukemias. Ongoing high-throughput sequencing initiatives have identified numerous solitary and recurring somatic point mutations within the various leukemic subtypes. The interpretation of this sequencing data requires the identification of driver versus passenger mutations. Driver mutations cause genetic alterations contributing to leukemic pathophysiology, whereas passenger mutations occur in leukemia cells and are propagated but are not etiologic to the disease.13 It is, therefore, essential that newly discovered somatic mutations in leukemia undergo subsequent biologic validation through sequencing studies in an experimental model system.
MUTATIONS THAT TARGET CORE-BINDING FACTOR
Core-binding factor (CBF) is targeted by more than a dozen different chromosomal translocations in acute leukemias, including the t(8;21) or inv(16), observed in approximately 20% of AMLs, and the t(12;21), present in approximately 25% of patients with pediatric B-lineage acute lymphoblastic leukemia (ALL).14 Adult patients with CBF leukemias have a favorable prognosis and the translocation ETS leukemia (TEL)/AML1 fusion that is expressed as a consequence of t(12;21) in children confers a favorable prognosis among B-cell ALL.15 CBF is a heterodimeric transcription factor composed of the AML1 (also known as RUNX1 or CBFA2) and CBFβ proteins that is critical for normal hematopoietic development. Loss of function of either subunit results in a complete lack of definitive hematopoiesis.16,17 The AML1 subunit of CBF contacts DNA but only weakly transactivates target genes as a monomer. When bound to its heterodimeric partner CBFβ, which does not itself contact DNA, transactivation of CBF target genes is dramatically enhanced.15,18,19 CBF transactivates a spectrum of target genes that are important in normal myeloid development, including transcription factors (e.g., PU.1, CEBP/A and GATA1), cytokines (e.g., granulocyte-macrophage colony-stimulating factor [GM-CSF]) and cytokine receptors (such as macrophage-colony stimulating factor [M-CSF] receptor), as well as in lymphoid development, such as the T-cell receptor beta (TCRβ) enhancer and the immunoglobulin (Ig) heavy-chain loci.18–21 Because CBF targets genes that are important for normal hematopoietic development, a mutation or gene rearrangement that resulted in loss of function of either AML1 or CBFβ might be expected to impair hematopoietic differentiation.15,18,19
In addition to frequent involvement of AML1 as a consequence of chromosomal translocations, it has been determined that loss-of-function mutations in AML1 are responsible for the inherited leukemia syndrome familial platelet disorder with propensity to develop AML (FPD/AML).22,23 Approximately 3% to 5% of sporadic cases of AML harbor loss-of-function mutations in AML1,22,24 with a higher frequency in M0 AML (25%) and in AML or myelodysplastic syndrome (MDS) with trisomy 21. AML1 loss-of-function mutations are associated with poor rather than good prognosis subgroups such as acute myeloid leukemia 1/eight twenty-one (AML1/ETO); however, this may be a result of occurring in the context of myelodysplasia or mixed lineage leukemias.19
Compelling evidence has been shown that translocations that target CBF result in a loss of function through dominant negative inhibition. The AML1/ETO fusion associated with t(8;21) and the CBFβ/MYH11 (aka CBFβ/SMMHC) fusion associated with inv(16) are dominant negative inhibitors of CBF and impair hematopoietic differentiation. The expression of either the AML1/ETO or CBFβ/MYH11 fusion genes from their endogenous promoter in mice completely inhibits the function of the residual AML1 or CBFβ alleles, resulting in a lack of definitive hematopoiesis and resultant embryonic lethality.16,17 The phenotype observed is the same as that seen in AML1–/– or CBFβ–/– mice, indicating that the AML1/ETO or CBFβ/MYH11 fusions, respectively, act as potent dominant negative inhibitors of the native proteins.25,26 However, AML1/ETO and CBFβ/MYH11 have been shown to confer novel contributory gain-of-function effects beyond those involving CBF transcriptional targets. For example, AML1/ETO blocks the tumor suppressors p14ARF and nuclear factor 1 (NF1), whereas CBFβ/MYH11 blocks the expression of p15INK4B.27,28 AML1/ETO downregulates DNA-repair genes, perhaps enhancing genomic instability.29 Histone deacetylases (HDAC) and DNA-methyltransferases (DNMT) associated with AML1/ETO alter the epigenetic profile of normal and leukemic cells to generate global changes in gene expression, which may be integral to leukemogenesis.30,31 Respective inhibitors such suberanilohydroxamic acid (SAHA) and azacytidine may, therefore, have therapeutic value in CBF leukemias.18
Although the expression of AML1/ETO leads to alterations of gene expression and hematopoietic cell proliferation leukemia and confers the ability to serially replate in methylcellulose culture (a measure of self-renewal potential), this does not result in the development of leukemia in an animal model. However, coexpression of an alternatively spliced isoform of the AML1/ETO transcript, AML1/ETO9a, which includes an extra exon, exon 9a, of the ETO gene (AML1/ETO9a encodes a C-terminally truncated AML1-ETO protein of 575 amino acids) leads to a rapid development of leukemia in a mouse retroviral transduction–transplantation model.32 The presence of AML1/ETO9a closely correlates with the presence of activating c-KIT mutations in humans, conferring a poor prognosis.33 Similarly, the expression of CBFβ/MYH11 in adult hematopoietic cells results in leukemia only after a markedly prolonged latency; this latency can be shortened using mutagenesis strategies.26 In summary, translocations that target CBF impair hematopoietic differentiation and confer certain properties of leukemic stem cells, such as the ability to serially replate, but are not sufficient to cause leukemia.
In pediatric B-ALL, 25% of cases have t(12;21)(p13;q13) in which the TEL (aka ETV6) gene translocates into AML1, thus allowing the production of a chimeric protein, TEL/AML1 (aka ETV6/RUNX1).34,35 TEL is a transcriptional repressor mediated through associated HDACs and, like AML1, has requirements in fetal and definitive hematopoiesis.30,34,36 TEL has 30 known fusion partners of different functional classes, including tyrosine kinases such as in TEL/platelet-derived growth factor receptor (PDGFR) and HOX genes such as in PAX5/TEL, although TEL/AML1 is the most common.34 The fusion protein preserves the central repressor domain in TEL and contains almost the entire AML1 protein sequence. TEL/AML1 has the ability to bind to AML1 consensus sequences but now brings an HDAC-dependent repressor function to AML1-responsive promoter elements, thereby suppressing AML1 targets.34 In addition, physiologic TEL function may be dysregulated by the fusion through heterodimerization via TEL helix–loop–helix domains.34 TEL/AML1 is often found in conjunction with deletions in the pre-B receptor, VPREB, and the tumor suppressor gene, CDKN2A.37
CHROMOSOMAL TRANSLOCATIONS THAT TARGET THE RETINOIC ACID RECEPTOR ALPHA GENE
The empiric observation that all-trans-retinoic acid (ATRA) induces complete responses in patients with acute promyelocytic leukemia (APL) drove the subsequent cloning of the t(15;17)(q22;q21) fusion gene involving the RARα locus. Several groups demonstrated at approximately the same time that the RARα gene on chromosome 17 was fused to a novel partner that was eventually identified as the promyelocytic leukemia (PML) gene.38–40 Two reciprocal fusion RNA species are produced as a consequence of the translocation, RARα/PML and PML/RARα. The PML/RAR-α fusion protein contains the zinc finger of PML fused to the DNA- and protein-binding domains of RAR-α. Several other chromosomal translocations target the RARα locus and are associated with an APL phenotype. The best studied of these is the promyelocytic leukemia zinc finger (PLZF/RAR-α) fusion, which also aberrantly recruits the nuclear corepressor complex. However, in contrast with the PML/RAR-α fusion, ATRA is not able to relieve corepression mediated by the PLZF/RAR-α fusion and thus is not effective in patients who harbor the t(11;17) associated with this fusion gene.41
The PML gene has a broad, although incompletely understood role in the homeostasis of nuclear proteins as an organizing component of nuclear bodies (PML-NB) responsible for nuclear structure and shuttling.42,43 Recently, an important independent cytoplasmic role for PML as a tumor suppressor has been uncovered, whereby PML localized to the contact points between the endoplasmic reticulum and mitochondrial-associated membranes controls calcium transport and apoptosis.44 PML is essential for maintaining self-renewal in hematopoietic stem cells (HSC) as shown by a mouse knockout model of PML, which demonstrated increased proliferation and premature exhaustion in the HSC compartment.45 The PML region of PML-RARα was also identified as the target for arsenic trioxide therapy. The binding of arsenic increases PML oligomerization followed by SUMOylation, which in turn, causes PML/RARα degradation.46
RARα possesses a DNA-binding domain, a hormone-binding domain, and a retinoid X receptor (RXR)–binding domain, all of which are included within the PML/RARα chimeric protein. RARα transactivates multiple genes involved in myeloid differentiation.47 PML/RARα homodimers, enabled via a coiled coil domain in the PML portion, bind to RAR sites and repress genes important for granulocytic differentiation in part through HDAC and corepressor recruitment.47 PML/RARα, however, does not have effects solely through dominant inhibition of RARα/RXR binding sites. PML/RARα was shown to have an extended repertoire of DNA consensus binding sites beyond those found for RARα.48 PML/RARα appears to extensively modify histone-acetylation and methylation marks in expressing cells in an ATRA-dependent manner, likely through recruitment of HDACs and histone methyltransferases and demethylases within the PML-RARα complex.48,49 Drug targeting of PML/RARα-associated epigenetic modifying proteins may, therefore, provide effective adjuncts to ATRA-based therapies.
The transforming properties of the PML/RARα fusion gene have been tested in murine models. Expression of PML/RAR-α in transgenic mice from promoters that direct expression to the promyelocyte compartment result in an APL-like phenotype.50–52 However, there is approximately a 6-month lag before the development of leukemia, incomplete penetrance of approximately 15% to 30%, and acquired karyotypic abnormalities, all suggesting that second mutations are required for induction of leukemia. In at least some cases, activating mutations in FLT3 may be the additional mutation required. ATRA is efficacious in leukemic animals expressing both PML/RAR-α and activated FLT3, and this model has allowed for the preclinical testing of newer agents such as arsenic trioxide.53
MUTATIONS THAT TARGET HOX FAMILY MEMBERS
HOX genes are homeodomain-containing transcription factors important in patterning in vertebrate development and in hematopoietic development.54,55 HOX genes are clustered in four genomic loci HOXA-D, although additional orphan HOX genes occur elsewhere in the genome. Transactivation by HOX genes is potentiated by cofactors such as pre–B cell leukemia (PBX1) and myeloid ecotropic insertion site (Meis1). NUP98, a nuclear transport protein, is a fusion partner to at least eight different HOX genes in AML as well as numerous other genes.56 HOX gene expression is tightly regulated during hematopoietic development. HOXA9, for example, is expressed in early hematopoietic progenitor cells but is downregulated during hematopoietic differentiation and is undetectable in terminally differentiated cells. The expression of NUP98/HOXA9 results in derepression of HOXA cluster genes, several of which promote HSC self-renewal.54,56
The contribution of the NUP98 moiety to leukemic transformation is not fully understood. NUP98 is normally a component of the nuclear pore complex and is constitutively and ubiquitously expressed. However, several lines of evidence suggest that NUP98 contributes more than a constitutively activated promoter. For example, NUP98 motifs known as FG repeats are essential for transformation and may serve to recruit transcriptional coactivators, such as CBP/p300, to HOXA9 DNA-binding sites.57 In murine models of leukemia, overexpression of HOXA9 alone is not sufficient to cause AML, but coexpression of HOXA9 with transcriptional cofactors, such as MEIS1, results in efficient induction of AML.58 Thus, the NUP98 moiety in the context of the NUP98/HOXA9 fusion may serve multiple functions, including provision of an active promoter, and recruitment of transcriptional coactivators such as CBP/p300 that subserve the function of other cofactors such as MEIS1. Epidemiologic evidence that the NUP98 moiety contributes to leukemogenesis includes the observation that there are now a spectrum of fusion proteins involving components of the nuclear pore that are targeted by chromosomal translocations in acute leukemias. These include NUP98 and NUP214 fused to a diverse group of partners, including HOXA9 and HOXD13, and the DDX10, PMX1, DEK, and ABL1 genes, respectively.
Dysregulated HOX gene expression may be important in leukemias that do not directly target HOX family members. Several proteins that are upstream of HOX expression have been observed as fusion genes associated with AML, the most frequent of these are MLL gene rearrangements. More than 40 chromosomal translocations target MLL and result in fusions of MLL with a broad spectrum of partners. In addition, dysregulation of HOX genes by an enhancer effect, which occurs in t(7;10)(q34;q24), where the TCRβ translocates into the HOX11 locus, is an important contributor to T cell acute lymphoid leukemia (T-ALL) leukemogenesis.59 However, a common biologic feature of all of these may be their ability to dysregulate HOX gene expression during hematopoietic development. For example, t(12;13) associated with AML results in the expression of high levels of CDX2 from the TEL locus.60 CDX2 is a homeotic protein that regulates the expression of HOX family members in the colonic epithelium. As in hematopoietic development, HOX gene expression is highest in colonic stem cells in the colonic crypts and is downregulated with maturation. It has been shown that CDX2 and CDX4 can dysregulate HOX expression in hematopoietic progenitors and can result in leukemia.58,60 Evidence to support this includes the ability of CDX2 to induce leukemia in murine retroviral transduction models.58,60
Taken together, these data indicate that the NUP98/HOXA9 fusion transforms hematopoietic progenitors in part through dysregulated overexpression and by transactivation mediated through the NUP98 transactivation domain that recruits CBP. However, like other gene rearrangements involving hematopoietic transcription factors, expression of NUP98/HOXA9 alone is not sufficient to cause leukemia. In murine bone marrow transplant models, NUP98/HOXA9 induces AML only after markedly prolonged latencies indicative of a requirement for second mutation. Coexpression of Meis1 or FLT3ITD with NUP98/HOXA9 in mice significantly shortens the latency; however, these mutations are not present in all human cases, suggesting that additional cooperating pathways exist.61,62
CHROMOSOMAL TRANSLOCATIONS THAT TARGET THE MLL GENE
The MLL locus is involved in more than 80 different chromosomal translocations with a remarkably diverse group of fusion partners63,64 and are associated with mostly French-American-British (FAB) subtype M4 or M5, and fewer with M2 AML. Patients who have received prior chemotherapy for cancer and develop AML (therapy-related MDS/therapy-related AML [t-AML]) often have abnormalities in 11q23, especially those patients treated with topoisomerase inhibitors such as etoposide or topotecan. Chromosomal translocations involving band 11q23 result in the expression of a fusion gene containing amino-terminal MLL sequences fused to a wide variety of partners. There has been no common functional motif or activity ascribed to all partners; however, specific fusions may be associated with specific leukemic phenotypes. The MLL/AF4 fusion associated with t(4;11) is frequently observed in infant leukemias and is associated with an ALL phenotype in more than 90% of cases, whereas the MLL/AF9 fusion associated with the t(9;11) is almost exclusively associated with AML. Certain MLL fusion genes also have prognostic significance. For example, patients with t(9;11)(p22;q23) have a better outcome than those with other translocations involving 11q23.
The MLL gene encodes a large, ubiquitously expressed protein. The Drosophila protein trithorax, a homolog of MLL, regulates patterning and HOX gene expression during development. It has been hypothesized, in part based on these observations, that MLL might be required for maintenance of HOX gene expression.65 Mice that have homozygous deficiency for MLL have an embryonic lethal phenotype at postconception day 10.5. Even heterozygous animals have developmental anomalies in the axial skeleton and hematopoietic deficits, including anemia.66 Thus, as for other genes targeted by chromosomal translocations, MLL is important for normal hematopoietic development.
Major advances have been made recently regarding the mechanisms underlying MLL and MLL-fusion gene function. MLL binds a broad cohort of epigenetic regulators including bromodomain-containing 3 and 4 (BRD3 and BRD4); the H3K79 histone methyl transferase, DOT1; the lysine-specific demethylase, KDM1A; and the polycomb-repressive complex 2 (PRC2). Although MLL possesses a Su(var)3-9, enhancer-of-zeste and trithorax (SET) homology H3K4 histone methyl-transferase, it is not retained in MLL-fusion proteins. MLL and MLL fusions also require complex formation with menin (MEN1) and lens epithelium–derived growth factor (LEDGF) to be able to interact with DNA and activate target genes.67,68 MLL fusions have been shown to affect transcriptional poising at target genes as part of a larger super elongation complex (SEC).69 The control of RNA polymerase II elongation after recruitment to promoter sites provides an additional layer of regulation to target genes such as HOXA9.69,70 MLL fusions also form a complex known as DotCom, which, via associated DOT1 activity, yields H3K79 di/trimethylation marks, thus altering expression at affected genes.69 MLL-fusion/DotCom interaction allows binding and transcription at target genes of the Wnt/β-catenin pathway via binding of nuclear-localized β-catenin. The Wnt pathway is essential for fetal HSC self-renewal, and aberrant activation of its downstream targets mediated by MLL-fusions is hypothesized to confer self-renewal properties to leukemic stem cells (LSCs).71
Although various MLL fusions have similar transforming properties in vitro, there are distinctive differences in disease penetrance and latency in the murine models depending on the fusion partner. It is possible that the MLL gene rearrangement may be critical for transformation, whereas the fusion partners confer properties related to disease phenotype. The long latency of disease in murine models supports the hypothesis that MLL fusions, like the PML/RAR-α and CBF-related fusion proteins, require second mutations to cause leukemia.
As noted previously in the section Leukemic Stem Cell, data indicate that certain MLL fusion genes may also confer properties of self-renewal to hematopoietic progenitors. MLL/ENL expression in common myeloid progenitors or granulocyte-monocyte progenitors in a murine system conferred properties of self-renewal, including the ability to serially replate in methylcellulose cultures and to engender a transplantable AML phenotype in recipient animals.5 Similarly, in a mouse model of MLL/AF9 oncogene-induced leukemia, up to a quarter of the leukemic cells exhibited stem cell behavior.72 Furthermore, the MLL/AF9-positive LSC are heterogeneous as they give rise to ALL when injected into immunodeficient mice. The same cells cause AML when injected into immunodeficient mice that are transgenic for the human genes stem cell factor (SCF), GM-CSF, and interleukin 3 (IL-3).73 These data indicate that leukemogenic mutations may occur in cells that have no intrinsic self-renewal capacity and yet confer these properties by activation of specific transcriptional programs, which may be further modified by clues from the microenvironment.
MUTATION OF C/EBPα
C/EBPα is a 42-kDa hematopoietic transcription factor that is required for normal myeloid lineage differentiation and that inhibits proliferation.74 C/EBPα is downregulated in 50% of AML, often through methylation of its promoter region.75 Therefore, loss of C/EBPα function in leukemia likely impairs myeloid differentiation and removes a block on proliferation.76 Two major types of C/EBPα point mutations have been described in AML—short frame-shifting mutations in the region encoding the amino-terminus, causing the expression of a shortened 30-kDa protein with dominant negative activity, and in-frame insertions or deletions in the region of the carboxy-terminus, which alter the DNA-binding or dimerization domains, causing a loss of function.77 Two-thirds of C/EBPα-mutated leukemias have both N- and C-terminal mutations on each allele.76 Although the bulk of C/EBPα mutations occur in patients with normal cytogenetics, overall and progression-free survival is more favorable; therefore, C/EBPα status is an important prognostic determinant.78 In contrast to AML, 2% of pre–B-ALL has been shown to have an upregulation of C/EBPα or family members through enhancer effects of IgH locus translocation into the C/EBPα locus as seen in t(14;19)(q32;q13).79,80
MUTATION OF GATA-1
GATA-1 mutations are associated with a subset of acute megakaryocytic leukemias (AMKL) (FAB M7), in particular leukemias arising in patients with Down syndrome (constitutional trisomy 21).81 GATA-1 is a transcription factor promoting erythroid and megakaryocytic development.82,83 GATA-1 mutations result in the early termination of the full-length GATA-1 protein; however, translation of a short-form (GATA-1s) from an alternate initiation codon occurs. GATA-1s is theorized to function as either a hypomorphic or dominant negative allele, and dysregulation of GATA-1 pathways are thought to contribute to leukemogenesis.84,85 GATA-1 mutations are often seen in a transient myeloproliferative disorder (TMD), which precedes Down syndrome–associated AMKL, suggesting that a GATA-1 mutation is an early event cooperating with germ-line trisomy 21.84 GATA-1 mutations have been noted in Down syndrome fetal livers, and GATA-1s expression in a mouse model causes hyperproliferation of fetal liver megakaryocytes, supporting the hypothesis of an in utero origin of the disease.86 Current efforts to identify the critical cooperating genes dysregulated in trisomy 21 are utilizing mouse models possessing trisomies syntenic with the 8.35-Mb Down syndrome critical region to identify candidate genes.87
t(1;22) TRANSLOCATION ASSOCIATED WITH INFANT AMKL
The t(1;22)(p13;q13) is associated with the majority of non–Down syndrome AMKL in infants and results in the expression of the OTT1/MAL (aka RBM15/MKL1) fusion gene.88,89 OTT1 (RBM15) contains three amino-terminal RNA recognition motifs and a Spen paralog and ortholog C-terminal motif that is a transcriptional activator/repressor. Ott1 deletion in mice reveals multiple hematopoietic roles, including megakaryocyte growth and hematopoietic stem cell function.90,91 The MAL (MKL1) gene is a Rho-GTPase–regulated cofactor for serum response factor (SRF) and controls megakaryocyte development.92,93 A knockin mouse model expressing OTT1/MAL is able to recapitulate AMKL and demonstrated constitutive transcriptional activation from recombination signal binding protein for immunoglobulin kappa J (RBPJκ) binding sites, including Notch1 downstream targets, which is essential for its pathogenesis.94
MUTATIONS OF EPIGENETIC MODIFIERS
Several translocations associated with leukemia involve transcriptional coactivators and chromatin modifying proteins that have no apparent DNA-binding specificity. These include the mixed-lineage leukemia/ CREB-binding protein (MLL/CBP) and MOZ/CBP fusions that involve the transcriptional coactivator CBP and the MLL/p300 and MOZ/TIF2 fusions, which involve the coactivators p300 and TIF2, respectively.95,96 Although TIF2 itself is not known to have histone acetylase transferase (HAT) activity, a hallmark of the coactivators CBP and p300, it has a well-characterized CBP interaction domain that serves to recruit CBP into a complex with MOZ/TIF2.97 Thus, recruitment of CBP/p300 is a shared theme among this group of fusion genes.
The transcriptional targets and transformation properties of this class of fusion proteins are not fully understood. Transduction of MLL/CBP into primary murine bone marrow cells followed by transplantation results in a long-latency AML, suggesting the need for secondary mutations.98 MOZ/TIF2 also results in leukemia in a similar model system. MOZ is a HAT protein that contains a nucleosome-binding domain and an acetyl–coenzyme A–binding catalytic domain. A mutational analysis shows that leukemogenic activity requires MOZ nucleosome-binding activity and CBP recruitment activity, but the MOZ HAT activity is dispensable. These data would be consistent with a CBP gain-of-function in which CBP is recruited to MOZ nucleosome-binding sites.97 However, it has also been hypothesized that the leukemogenic potential of this class of fusions may be related to dominant negative interference with CBP/p300 or that the translocation leads to simple loss of function of CBP expressed from one allele. In support of this hypothesis, loss of a single allele of CBP/p300 in the human Rubinstein-Taybi syndrome increases predisposition to malignancies, including colon cancer and mice that are heterozygous for CBP that develop hematopoietic tumors.99
The TET2 gene located on 4q24 belongs to the ten-eleven translocation (TET) family (TET1, TET2, TET3), which converts 5-methylcytosine (5-mC) to hydroxymethylcytosine (5-hmC), the initial step in DNA demethylation. Mutations of the TET2 gene have been found in 8% to 27% of AML cases, in 20% to 25% of MDS cases, and in 4% to 13% of myeloproliferative neoplasms (MPN) cases. TET2 mutations are mono-allelic loss of function in most cases, including missense, frameshift, and nonsense mutations. The presence of the mutant TET2 is associated with superior survival in MDS and inferior survival in AML and chronic myelomonocytic leukemia (CMML).100 TET2 mutations are almost mutually exclusive with isocitrate dehydrogenase (IDH1/2) mutations, suggesting a similar epigenetic defect as IDH1/2 mutations.101 In vivo, TET2 inactivation induced both myeloid and also lymphoid malignancies. The precise mechanisms and downstream effects of TET2 are as yet unknown.102
Mutations in the gene encoding IDH1/2 functionally overlap with TET2 mutations, resulting in hypermethylation of leukemia cells, disruption of TET2 function, and impaired hematopoietic differentiation. IDH1 and IDH2 are nicotinamide adenine dinucleotide phosphate (NADP)-dependent IDHs that catalyze isocitrate to alpha-ketoglutarate (α-KG) in the tricarboxylic acid (TCA) cycle.103 IDH1/2 mutations are detected in 15% to 33% of AML, mostly in normal karyotype AML, in 3.5% of MDS, in 2% to 5% of MPN, and also in glioma.103 The mutations have been shown to exhibit a gain of function, leading to an aberrant accumulation of 2-hydroxyglutarate (2-HG). 2-HG is an oncometabolite, which inhibits an enzymatic activity of TET2 and stimulates hypoxia-inducible factor 1-alpha (HIF1α), leading to the initiation and promotion of cancer.104
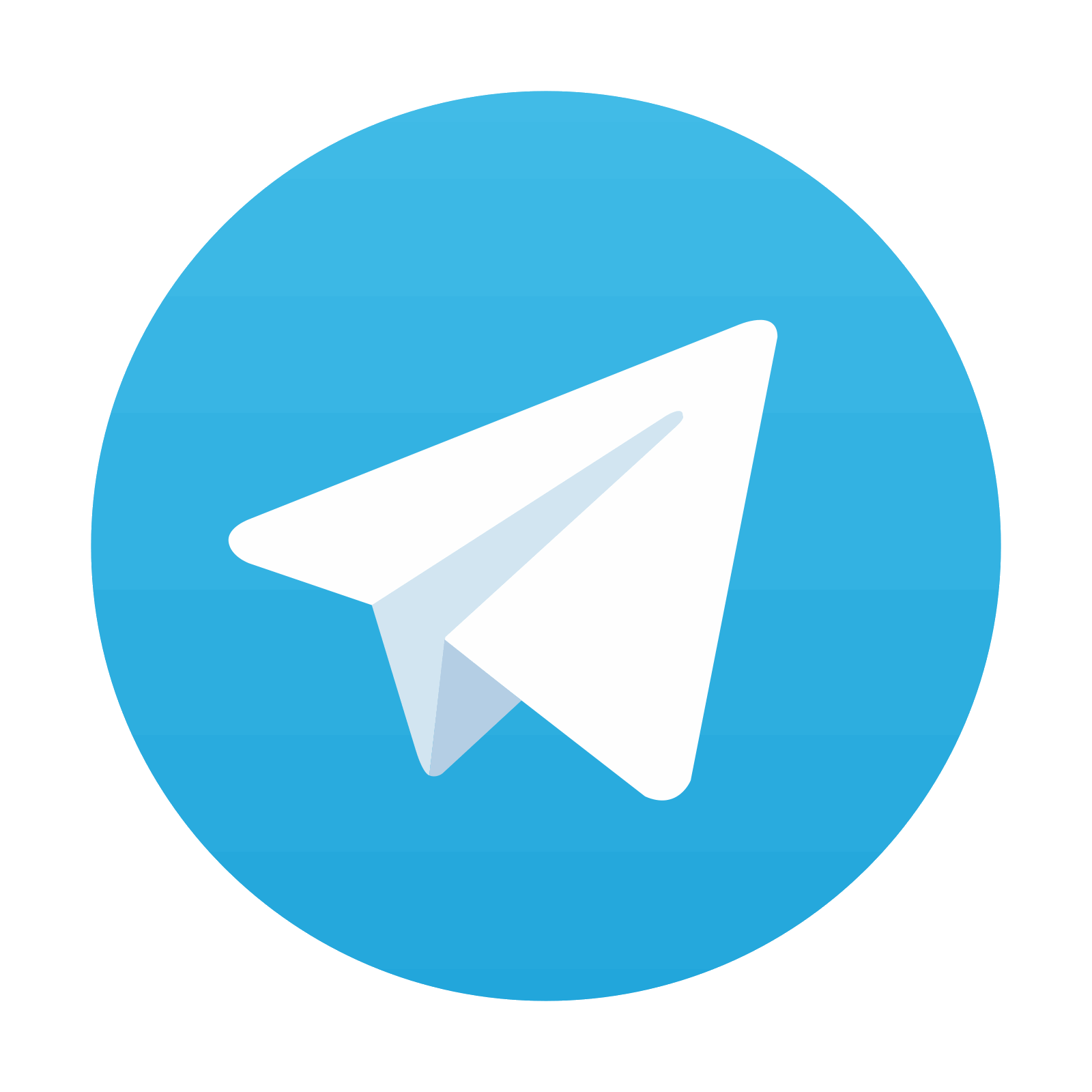
Stay updated, free articles. Join our Telegram channel
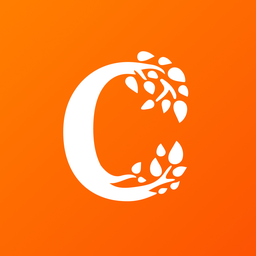
Full access? Get Clinical Tree
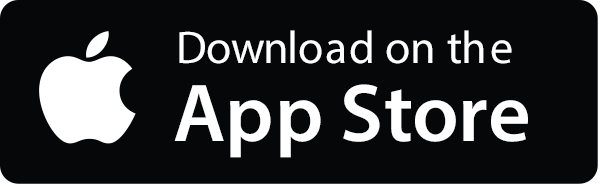
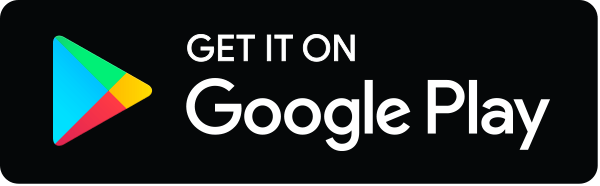