© Springer-Verlag Berlin Heidelberg 2017
Sun-Whe Kim and Hiroki Yamaue (eds.)Pancreatic Cancer10.1007/978-3-662-47181-4_22. Molecular Alterations in Pancreatic Cancer
(1)
Institute for Integrated Medical Sciences, Tokyo Women’s Medical University, Tokyo, Japan
2.1 Introduction
Cancer has somatically mutated genes that contribute to outdrive cellular proliferation and formation of a tumor. To know what genes are mutated, DNA sequencing is necessary; however until recently, only a limited number of genes or portions of genes could have been analyzed in a routine laboratory practice because of a limited ability of DNA sequencing in time and cost. However, there emerged a game changer, the next-generation sequencer (NGS), in 2009, which brought a paradigm shift in a way of genetic studies of diseases [1]. When the human genome project was conducted, 1987–2003, the automated Sanger sequencer was a main tool for analyzing DNA sequence. While the Sanger sequencer, still considered to be the most accurate sequencer, can output ~250 k bases per day, NGS can output 750G bases/3 days, in which the difference between them is one million times. The human genome is consisted of 3G base pairs of DNA; therefore, the output of NGS corresponds to 250 times of the human haploid genome. Despite this enormous output, NGS is an error-prone sequencer that needs redundant reads, usually 100 times, to get accurate sequencing. Nevertheless, NGS has given a revolutionary impact on sequencing studies primarily because it enables to sequence individual human genome in an affordable cost in a few days in a single laboratory. Alterations in protein-coding genes can be analyzed by exome that sequences every exon of human genes. Exons span 45M bases in total in the human genome, so that the exome analysis enables much cost- and time-saving studies than the whole-genome analysis. Moreover, base alterations in exomes can be interpreted directly as nonsynonymous or synonymous mutations, which is much more straightforward than interpreting variations in noncoding regions of the genome. For analysis of somatic mutations in cancer cells, comparison of sequencing data between cancer cells and normal cells is necessary; therefore, careful sampling with distinguishing between cancer cells and normal cells is needed.
An exome analysis of the pancreatic cancer has firstly reported by Jones et al. in 2008 [2]. Although this study was by means of Sanger sequencing, they analyzed 24 cases of pancreatic ductal adenocarcinoma (PDA). By NGS, Biankin et al., a team of international collaboration under International Cancer Genome Consortium, published a result of exome combined with a copy number variation (CNV) analysis of 142 PDAs in 2012 [3]. Later they published a result of whole-genome analysis of 100 PDAs in 2015 [4], and a result of integrated analysis consisted of whole-genome, CNV, transcriptome, and methylome analysis of 456 PDAs in 2016 [5]. There also are some independent studies published elsewhere including one by Wang et al. regarding exome analyses of 15 PDA cell lines in 2012 [6] and the other by Witkiewicz et al. regarding exome analysis of 109 PDAs in 2015 [7].
2.2 Genetic Alterations in PDAs
Exome analyses of PDAs indicate that one PDA has approximately 60 nonsynonymous mutations in average [3, 4]. PDAs with microsatellite instability that is caused by mismatch repair deficiency have more than 100 mutations/case, often 500 [4]. Spectra of mutations show that C:G>T:A transition is enriched in PDAs, especially CpG>TpG mutations are common, which indicates that an aberrant methylation may be a major cause of the mutations [4]. Smokers usually show enrichment of C:G>A:T transversion, which is observed in a fraction of PDAs. On the other hand, enrichment of C>T transition in TpCpW site, which is caused by apolipoprotein B mRNA editing enzyme, catalytic polypeptide-like (APOBEC), a cytidine deaminase, is not common in PDAs [4].
Through the exome and CNV analyses, four commonly altered genes in PDAs, namely, KRAS, TP53, CDKN2A, and SMAD4, have reemerged [2–4, 7]. These four genes are altered frequently, 90% of PDAs harbor gain-of-function mutations in KRAS, 90% harbor mutations or loss of CDKN2A, 75% harbor TP53 mutations, and 50% harbor mutations or loss of SMAD4, which has been well known since the late 1980s; hence, NGS analyses have reconfirmed alterations of these “Big 4” genes in PDAs. Next commonly altered genes are those encoding proteins involved in chromatin regulation, i.e., KDM6A, KMT2C/MLL3, KMT2D/MLL2, ARID1A, ARID2, and PBRM1, which are altered in ~25% of PDAs [4, 5]. Other genes each altered in ~10% of PDAs are those functioning in DNA repair system including BRCA1, BRCA2, PALB2, and ATM; those functioning in RNA processing and/or splicing including SF3A1, SF3B1, U2AF1, U2AF2, RBM6, and RBM10; those functioning in wingless-type MMTV integration site (Wnt) pathway including CTNNB1; those functioning in transforming growth factor beta (TGFb) pathway including SMAD3, TGFBR1, TFGBR2, ACVR1B, and ACVR2A; and those functioning in phosphatidylinositol 3-kinase (PI3K) pathway including PIK3CA and PTEN [4, 5].
2.3 KRAS and the Mitogen-Activated Protein Kinase Pathway
KRAS encodes Kirsten rat sarcoma viral oncogene homologue (KRAS) that is a small guanosine triphosphate (GTP)-binding protein and one of three members of rat sarcoma viral oncogene homologues (RAS) [8]. KRAS is mutated in 90% of PDAs, and mutations are commonly observed in codon 12, 13, and 61 as missense mutations, in which G12D and G12V are most common [5, 9]. G12C mutations that are known to be common in lung cancer and recently shown as a specific druggable target [10] are not common in PDAs. RAS plays a central role in controlling of activities of numerous signal transduction pathways, most notably of the mitogen-activated protein kinase (MAPK) pathway. In the RAS-MAPK pathway, a membrane-bound enzyme-linked receptor, e.g., the epidermal growth factor receptor or the platelet-derived growth factor receptor, activated by ligand binding activates a guanine nucleotide exchanging factor that facilitates exchanging guanosine diphosphate (GDP) bound to RAS with GTP, which makes RAS activated. The GTP-bound RAS activates the MAPK cascade consisted of the mitogen-activated protein kinase kinase kinase (MAP3K)/V-Raf oncogene homologue (RAF), the mitogen-activated protein kinase kinase (MAP2K)/MAP kinase-ERK kinase (MEK), and the mitogen-activated protein kinase 1 (MAPK1)/extracellular signal-regulated kinase (ERK), in which an activated signal is passed on by sequential phosphorylating reactions. RAS has an intrinsic hydrolase activity that turns bound GTP to GDP, which inactivates itself; however, the mutations in KRAS cause decreasing of the hydrolase activity and, hence, protracting activity of itself as well as downstream signal transduction pathways. Active MAPK1/ERK translocates into nucleus and activates transcription factors that induce expression of effector genes functioning in DNA replication, RNA maintenance, transcription and translation, cell cycle and mitosis, transporting, and cell proliferation [11]. Activity of MAPK1/ERK is negatively regulated by dual specificity phosphatases (DUSPs), most directly by DUSP6 [12]. DUSP6 forms a negative feedback loop with MAPK1/ERK, i.e., an activation of MAPK1/ERK induces expression of DUSP6 that inactivates MAPK1/ERK; therefore, MAPK1/ERK activity is tightly regulated through this negative feedback loop [13]. However in some PDAs, expression of DUSP6 is downregulated mostly by aberrant methylation; hence, the negative feedback loop between MAPK1/ERK and DUSP6 is abrogated, which results in constitutive activation of MAPK1/ERK and expression of genes implicated in malignant phenotypes of PDAs [14].
BRAF encodes B-Raf proto-oncogene, serine/threonine kinase that functions as a MAP3K in MAPK pathway. BRAF is mutated in some of PDAs that harbor the wild-type KRAS; therefore, mutations in BRAF and KRAS are mutually exclusive in PDAs [15]. Most mutations of BRAF in human cancers including PDAs are observed as a V600E mutation, which turns the kinase constitutively active [16]. Vemurafenib is developed to target cancers with the BRAF V600E mutation [17].
2.4 TP53
TP53 encodes p53, a transcription factor involved in DNA damage response [18]. DNA damage provoked by irradiation and/or reactive oxygen species is sensed by and activates the ataxia telangiectasia mutated (ATM), a serine/threonine protein kinase, that phosphorylates p53. The phosphorylated p53 dissociates from the mouse double minute 2 homologue (MDM2), an E3-ubiquitin ligase, and binds DNA to induce expression of target genes that have a consensus binding sequence in their promoters. Most of these target genes of p53 encode proteins involved in cell cycle arrest, DNA repair, and apoptosis including p21, p27, BAX, PUMA, etc., which plays an important role in determining cells’ fate whether they survive through DNA repair or die by apoptosis to avoid accumulation of mutations caused by DNA damage [19]. Mutations in TP53 are found in 60–75% of PDAs. Mutations in TP53 are either frameshift mutations or missense mutations within a DNA-binding domain of p53. The missense mutation in p53 abrogates DNA-binding activity, which results in dysfunction of its transcription-activating activity. These mutations have been thought as loss-of-function mutations, which definitely is the case in frameshift mutations; however, a recent investigation indicates that missense mutations in p53, at least some of them, can modulate functions of chromatin remodeling proteins and then enhance transcriptions of certain genes that promote malignant phenotypes of cancer, which indeed are gain-of-function mutations [20]. p53 proteins with missense mutations are refractory to MDM2-mediated proteasomal destruction and, therefore, accumulate in the nucleus and appeared as overexpressed by immunohistochemistry [21].
2.5 CDKN2A
CDKN2A encodes the cyclin-dependent kinase inhibitor 2A (CDKN2A)/p16. CDKN2A/p16 plays a role in attenuation of cell cycle progression from G1 phase to S phase. For progression of the cell cycle, the cyclin-dependent kinase 4 (CDK4) is activated by binding with cyclin D; subsequently the activated CDK4 phosphorylates retinoblastoma protein (RB), and then, the phosphorylated RB dissociates from the E2F transcription factor 1 (E2F1), which facilitates nuclear translocation of E2F1 and expression of target genes necessary for the cell cycle progression [22]. CDKN2A inhibits the activation of CDK4 by hampering its binding to cyclin D and, therefore, attenuates cell cycle progression. CDKN2A is mutated and deleted homozygously in 25% and 10% of PDAs, respectively [4]. Moreover, CDKN2A is epigenetically silenced by aberrant hypermethylation in 50–60% of PDAs; hence, CDKN2A is functionally disrupted in almost all PDAs, which presumably contributes to uncontrolled cell cycle progression [23].
2.6 SMAD4
SMAD4 encodes the Sma and Mad protein homologue 4 (SMAD4). SMAD4 plays a role in signal transduction of the transforming growth factor β (TGFβ) pathway. TGFβ, a ligand, binds and facilitates formation of a heterodimer between TGFβ receptor (TGFBR) type 1 and TGFBR type 2 and, then, induces phosphorylation of the receptors at each other. The phosphorylated TGFBR type 1 recruits and phosphorylates the Sma and Mad protein homologue 2 (SMAD2) or the Sma and Mad protein homologue 3 (SMAD3). The phosphorylated SMAD2/SMAD3 oligomerizes with SMAD4, and the SMAD2/SMAD3-SMAD4 oligomer translocates into nucleus and activates transcription factors, which induces expression of target genes that harbor TGFβ-responsive element in their promoters. The target genes include MMP3 and ADAM19, which encode matrix metalloproteinases; ARHGAP5 and ARHGAP10, which encode Rho-GTPase-activating proteins; CSNK1A1, DKK1, and FRAT1, which encode proteins associated with Wnt pathway; CASP8 that encodes a caspase; HDAC9 that encodes a histone deacetylase; and CAMK2D that encodes a protein associated with calcium signaling [24]. SMAD4 is mutated and homozygously deleted in around 20% and 10% of PDAs, respectively [4, 25]. Many of the mutations are nonsense or frameshift mutations, which indicates that SMAD4 alterations in PDAs are virtually loss-of-function alterations. SMAD4 alterations in PDAs can be detected by immunohistochemistry as complete loss of its protein product in cancer cells [21].
2.7 Chromatin Modification Genes
Chromatin remodeling genes, KDM6A, KMT2C/MLL3, KMT2D/MLL2, ARID1A, ARID2, and PBRM1, are altered in ~25% of PDAs. KDM6A/UTX encodes the lysine-specific demethylase 6A (KDM6A) that contains a Jumonji C domain and catalyzes the demethylation of tri-/dimethylated histone H3 [26]. KMT2C/MLL3 and KMT2D/MLL2 encode the lysine-specific histone methyltransferase 2C (KMT2C) and the lysine-specific histone methyltransferase 2D (KMT2D), respectively, which are members of the ASC-2/NCOA6 complex (ASCOM) that are involved in transcriptional coactivation [27, 28]. Because KDM6A is associated with KMT2C and KMT2D, alterations of KDM6A, KMT2C, and KMT2D are mutually exclusive [5]. ARID1A encodes the AT-rich interactive domain 1A (SWI-like), a member of the SWI/SNF family, whose members have helicase and ATPase activities and are able to restructure the nucleosome to make its DNA accessible during transcription, replication, and DNA repair [29]. ARID2 encodes the AT-rich interactive domain 2/BAF200, an integral component of the polybromo-associated BAF (PBAF) chromatin remodeling complex of the SWI/SNF family, which facilitates a ligand-dependent transcriptional activation by nuclear receptors [30]. PBRM1 encodes the polybromo 1/BAF180 that is another subunit of PBAF complex [31]. ARID2/BAF200 and PBRM1/BAF180 are mutually exclusive in constitution of the PBAF complex, which contributes to distinct selection of remodeled genetic elements [30]. Mutations of these genes are largely loss-of-function mutations, which is thought to cause dysfunction of chromatin regulation and aberrant expression of target genes although detail of dysfunction of these molecules in PDA is waited to be elucidated [5].
2.8 DNA Repair-Associated Genes
DNA repair-associated genes including BRCA1, BRCA2, PALB2, and ATM are altered in ~10% of PDAs. BRCA1 and BRCA2 encode the breast cancer 1 (BRCA1) and the breast cancer 2 (BRCA2), respectively, both of which are involved in repair of a double-strand break of DNA by homologous recombination. When DNA suffers from a double-strand break caused by ionizing radiation and/or reactive oxygen species, BACA1 associates with a broken end and helps to process the end being ready for the homologous recombination [32]. BRCA2 binds a single-strand DNA and helps to bring RAD51, a RecA homologue in eukaryotes, and plays a vital role in strand invasion in the homologous recombination, to damaging sites in DNA for its proper function [33]. ATM encodes the ataxia telangiectasia mutated (ATM) that is a kinase and functions in sensing of a DNA damage. ATM associates with a damaged site and phosphorylates some proteins including the checkpoint kinase 1 (CHK1) and the checkpoint kinase 2 (CHK2), which eventually results in cell cycle arrest for DNA repair. PALB2 encodes the partner and localizer of BRCA2 (PALB2) that, as this name suggests, is co-expressed with BRCA2 in nuclear foci when cells are irradiated, which indicates that PALB2 also participats in DNA repair [34]. Since most of mutations of these genes are loss-of-function mutations, a proper repair of DNA cannot be pursued, and, therefore, secondary mutations accumulate in cells with mutations of these genes.
2.9 RNA Processing Genes
Genes encoding RNA processing and/or splicing factors including SF3A1, SF3B1, U2AF1, U2AF2, RBM6, and RBM10 are mutated in ~16% of PDAs [5]. SF3A1, SF3B1, U2AF1, and U2AF2 encode components of U2AF, a small nuclear ribonucleoprotein (snRNP) essential for proper splicing of pre-messenger RNA. Most of mutations in these genes are missense mutations in a functional domain and are demonstrated to function as a dominant negative protein that facilitates immature splicing [35]. RBM6 and RBM10 encode RNA-binding molecules implicated in alternative splicing. Mutations of these genes are mostly loss-of-function mutations, which is demonstrated to be implicated in dysfunction of alternative splicing of some key molecules for oncogenesis like NUMB [36].
2.10 Wnt Pathway Genes
Wnt pathway is an important signaling pathway in development of multicellular organisms. One of important mediators in Wnt pathway implicated in pancreatic cancer is beta catenin. The gene encoding beta catenin is CTNNB1, which is mutated in ~10% of PDAs. Beta catenin is a cadherin-associated protein in the adherence complex that mediates cell-cell junction. Beta catenin is also a cytoplasmic protein that can function as a signal mediator, which is tightly regulated through formation of a complex with axin and the adenomatous polyposis coli (APC) protein. The complex of beta catenin-axin-APC associates with glycogen synthase kinases that phosphorylate and render beta catenin for ubiquitin-proteosomal destruction. Wnt signaling suppresses the complex formation and facilitates free beta catenin. The free beta catenin translocates into the nucleus and functions as a transcriptional coactivator [37]. Mutations in CTNNB1 cause to generate a protein refractory to the ubiquitin-mediated destruction, which results in facilitation of the transcriptional coactivator activity of beta catenin [38]. By immunohistochemistry, a mutated beta catenin is often found as an overexpressed protein in the nucleus.
2.11 Phosphatidylinositol 3-Kinase (PI3K) Pathway Genes
Genes encoding molecules implicated in PI3K pathway including PIK3CA and PTEN are mutated in ~5% of PDAs. PI3K pathway plays a fundamental role in cell growth. Phosphatidylinositol (PI) is a glycerophospholipid molecule sitting in the cell membrane. PI is phosphorylated by kinases on specific hydroxyl groups, PI-3, PI-4, and PI-5, and functions as a signal mediator. PIK3CA encodes the phosphatidylinositol-4,5-bisphosphate 3-kinase, catalytic subunit alpha. Mutations in PIK3CA mostly affect codon 545 or 1047, which causes upregulation of kinase activity of its protein product. PTEN encodes the phosphatase and tensin homologue, a phosphatase specific for PI-3. Mutations in PTEN are mostly frameshift or nonsense mutations that result in loss of function of its product.
2.12 Molecular Mechanism of Development of PDA
Pancreatic cancer has been hypothesized to develop from ductal cells, in which normal ductal cells would give rise to full-brown cancer cells via gradual dysplastic changes of themselves. PDA tissues usually have dysplastic ductal lesions in close vicinity of invasive carcinoma [39, 40], and studies on these dysplastic lesions have elucidated that dysplastic cells have varying grades of atypia, from low-grade to high-grade, and the high-grade lesions can be found frequently in a pancreatic tissue specimen with invasive cancer while extremely infrequently in that without invasive cancer [40]. Molecular studies have uncovered that low-grade dysplastic cells harbor KRAS mutations and CDKN2A inactivation, while high-grade dysplastic cells have aberrations of TP53 and SMAD4 in addition to the KRAS mutation and CDKN2A inactivation [21]. These results indicate that molecular aberrations indeed accumulate along with the progression of dysplastic grade of ductal cells. Now these dysplastic lesions are termed pancreatic intraepithelial neoplasia (PanIN) [41], i.e., PanIN is a noninvasive proliferative lesion of dysplastic ductal cells, which develops as a low-grade lesion and progresses to a high-grade lesion with an association with molecular alterations. This multistep hypothesis is known as the progression model for the pancreatic cancer [42].
The progression model for the pancreatic cancer seems to be further endorsed by studies of genetically engineered mouse models (GEMM) of pancreatic cancer. GEMM with lox-Stop-lox (LSL)-Kras G12D and Pdx1-Cre induces the mutant Kras, Kras G12D , the most common type of mutant KRAS in human PDAs, in a pancreas-specific manner during development. This mouse is born without any abnormality; however, it gradually develops microscopic proliferative lesions in the pancreatic duct that closely mimic PanIN and, eventually, although rarely, invasive ductal carcinoma in the pancreas in 2 years [43]. Detailed examinations of this model indicate that PanINs in early phase are with low-grade dysplasia, while those in late phase harbor high-grade dysplasia. GEMM with pancreas-specific expression of Kras G12D and Trp53 R172H (mutant p53) shows facilitation of development of invasive ductal carcinoma with frequent metastasis, which indicates that an accumulation of genetic alterations, the mutated Kras and the mutated Trp53 in this case, accelerates pancreatic cancer development [44]. Moreover, GEMM with pancreas-specific expression of Kras G12D and Trp53 R172H and complete loss of Smad4 develops a rapidly growing pancreatic tumor with metastasis and shows shorter survival than a mouse with Kras G12D and Trp53 R172H , in which overexpression of Runx3 is associated with the metastatic phenotype [45].
2.13 Genetic Alterations in Intraductal Papillary Mucinous Neoplasms
Intraductal papillary mucinous neoplasms (IPMNs) are characterized by manifestation of dilated duct filled with mucin. The dilated duct is lined with neoplastic cells growing in papillae with varying grades of atypia [46]. The papillae show diverse architectural variations termed gastric, intestinal, pancreatobiliary, and oncocytic subtypes [47]. The atypia ranges from low-grade to high-grade, which often intermingles with each other. Occasionally the neoplastic cells invade into parenchyma, which forms invasive mucinous colloid carcinoma or invasive ductal adenocarcinoma [48]. These features of IPMNs are quite distinctive from PDAs, a conventional type of pancreatic cancer; however, molecular alterations specific for IPMNs had been unknown until just recently. In 2011, two groups of researchers independently reported that IPMNs exclusively harbored somatic mutations in GNAS, which has uncovered a specific molecular pathway implicated in IPMNs [49, 50]. Somatic mutations in GNAS are observed in 50–70% of IPMNs while, strikingly, none of PDAs examined. GNAS encodes the guanine nucleotide-binding protein (G protein) stimulating alpha subunit (Gsα) that functions as a mediator in the G protein-coupled receptor (GPCR) pathway. GDP-bound Gsα forms a heterotrimeric G protein complex with β and γ subunits in its inactive state. A ligand binding to GPCR activates the guanine nucleotide exchanging factor that mediates exchange of GDP with GTP-bound Gsα. GTP-bound Gsα turns into an active form, dissociates from β and γ subunits, and subsequently associates with and activates adenylyl cyclase. The adenylyl cyclase mediates production of cyclic AMP, which leads to activation of the cyclic AMP-dependent protein kinase (PKA). PKA translocates into the nucleus and phosphorylates downstream molecules implicated in gene expression. Gsα has an intrinsic hydrolase activity that catalyzes the hydrolysis of the bound GTP to GDP, which inactivates itself. Mutations in GNAS observed in IPMNs almost always involve codon 201, which commonly are R201H or R201C. These mutations abrogate the intrinsic hydrolase activity, which results in constitutive activation of Gsα, hence, gain-of-function mutations [51]. An in vitro experiment to examine an effect of the mutant Gsα shows that transfection of mutated GNAS in pancreatic ductal cells induces elevation of cyclic AMP and marked alteration of gene expression including upregulation of mucin genes, which indicates that the mutated GNAS is strongly associated with production of excess mucin in IPMNs [52]. To examine an in vivo effect of the mutated GNAS, a genetically engineered mouse model that harbors LSL-GNAS R201H under CAG promoter (Tg(CAG-LSL-GNAS R201H )) was generated [53]. When this mouse crosses with Ptf1a Cre/+ and LSL-Kras G12D mice, synergistic expression of GNAS R201H and Kras G12D is induced in a pancreas-specific manner, and, as a result, a multicystic tumor develops in the pancreas within 5 weeks. The multicystic tumor is consisted of dilated ducts lined with papillary neoplastic epithelia, which closely mimics human IPMNs. This result indicates that the mutated GNAS indeed causes development of IPMN in vivo. GNAS mutations are observed in low-grade IPMNs as well as high-grade IPMNs [50]. In IPMN variations, intestinal-type IPMNs are more likely to harbor GNAS mutations than other types of IPMNs [54]. The pyloric gland variant of gastric-type IPMNs also commonly harbors GNAS mutations [55]. By immunohistochemistry, IPMN cells show strong expression of Gsα and phosphorylated substrates of PKA [50]. GNAS mutations are not associated with patients’ survival [54]. These observations indicate that the GNAS mutation strongly contributes to development and manifestation of characteristic phenotypes of IPMNs.
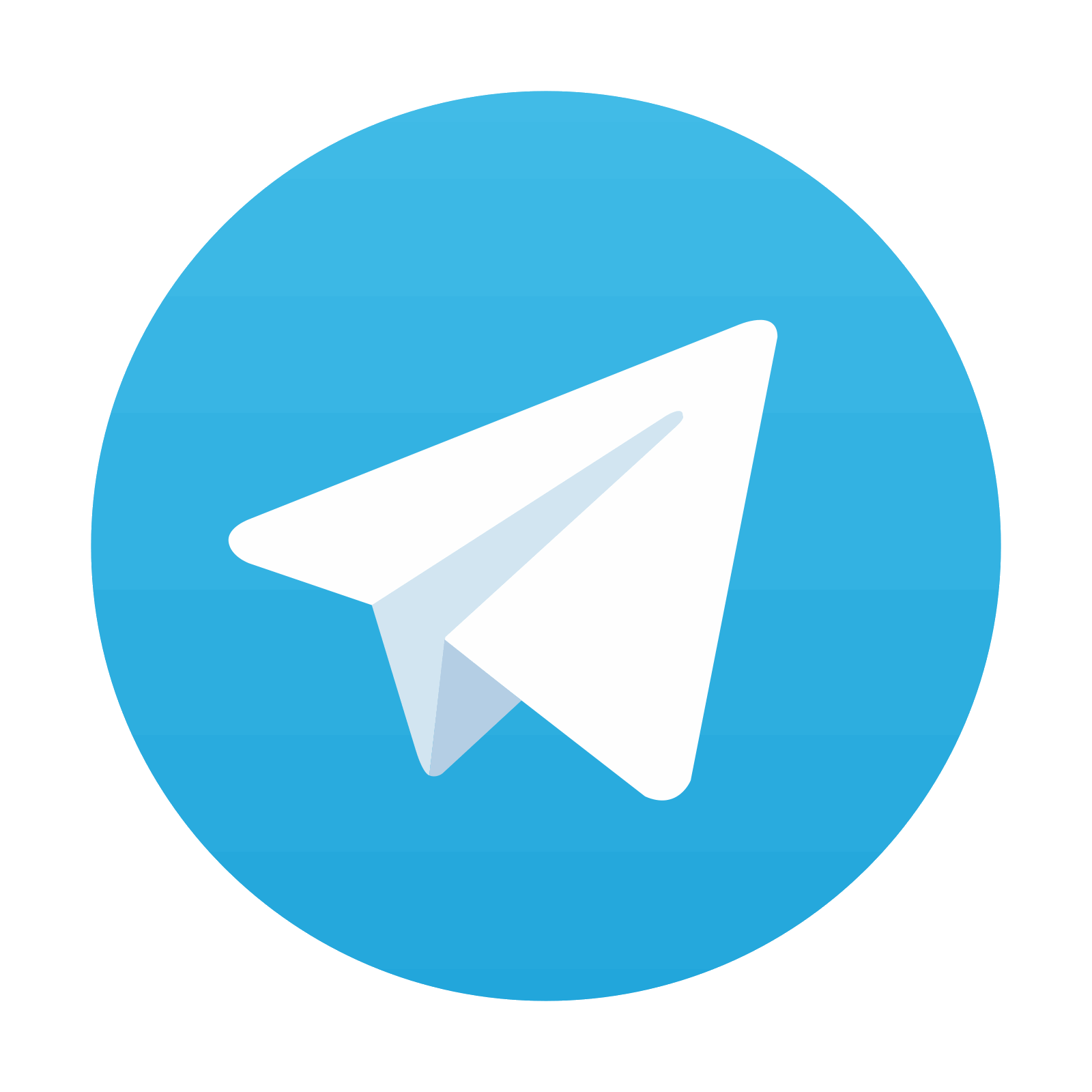
Stay updated, free articles. Join our Telegram channel
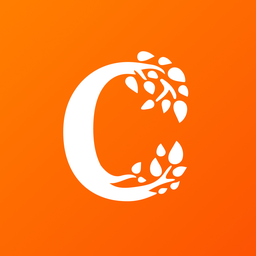
Full access? Get Clinical Tree
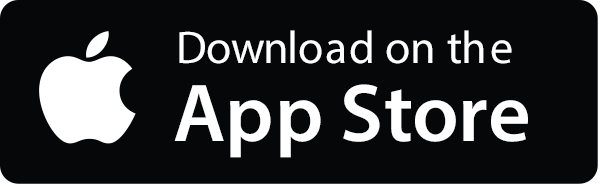
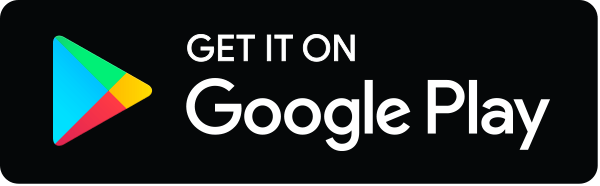