Fig. 4.1
Human germinal vesicle (a) and metaphase I (b) oocytes. Mitochondria are absent from the cortical region of the cytoplasm in germinal vesicle oocytes while they are dispersed in the ooplasm of metaphase I oocytes. Magnification × 5000; scale bar = 5 μm
In pronuclear stage of ova, mitochondria form an aggregated structure in the central region of the ooplasm around the pronuclei (PN). This occurs in normal and abnormal embryos. The structure and intracellular distribution of mitochondria in 2–8 cell embryos are similar to those in mature oocytes [26]. Although the density of mitochondria decreases slightly at the morula stage, the structure of their cristae remains unchanged as compared with those found in early stage embryos. Developing clear areas in mitochondrial matrices have been postulated to be the sites of localization of mitochondrial DNA and initiation of their transcription [19].
The most striking change in mitochondrial morphology occurs during the expansion and hatching of blastocysts. Round- or oval-shaped mitochondria undergo transformation into elongated tubular forms. The inner membranes of these mitochondria are enriched with well-defined transverse cristae, a morphological sign of increased metabolic activity. Extremely elongated mitochondria align parallel to the surface in stretching trophoblast cells that appear during blastocyst expansion [26]. Taken together, mitochondrial structure (oval shape with minimum amounts of cristae), but not their subcellular localization, remains unchanged during the maturation of oocytes from the GV stage to MI, MII, pronuclear embryo, and to the morula stage.
Mechanism of Mitochondrial Traffic in Mammalian Oocytes
The intracellular localization of mitochondria changes significantly during meiotic maturation of mammalian oocytes. Although the mechanism and molecular properties of mitochondrial traffic have been energetically studied in somatic cells, limited information is available about the dynamic aspects of this organelle in mammalian oocytes. Among the various somatic cells, mitochondrial traffic has been studied most extensively using cultured neuronal cells [33]. Because neuronal cells have characteristic long axons and cell bodies, bidirectional movement of mitochondria (and other organelles) either from cell bodies (minus end of microtubules) toward free nerve endings (plus end of microtubules; anterograde traffic) or from nerve endings toward cell bodies (retrograde or centripetal traffic) in cultured live cells can be observed precisely using a light microscope equipped with time-laps cinematography. Kinetic analysis revealed that mitochondrial traffic is closely associated with the cytoskeleton, such as microtubules and microfilaments [33, 34]. Mitochondrial movement in axons depends predominantly on microtubules, kinesin and dynein, motor protein families [33, 35–41]. Kinesins transport mitochondria and other organelles from the minus end to the plus end of microtubules. Among various motor proteins, Kif5b and Kif1b (class-1 and 3 kinesins, respectively) play critical roles in the mechanism of mitochondrial traffic in neurons. Knockout of Kif5b and Kif1b markedly changed mitochondrial distribution and energy metabolism in neurons [36, 38, 41]. Dyneins transport mitochondria and other organelles in neurons from nerve endings to cell bodies via centripetal traffic. The dynein heavy chain and related proteins have been shown to interact with mitochondria and other organelles [39]. Inhibition of dynein significantly altered the movement and distribution of mitochondria in axons [35, 37, 40].
It should be noted that neuronal cells survive for a long time in vitro without undergoing mitosis while most cultured somatic cells show active mitosis. It has been well documented that the intracellular localization and metabolic status of mitochondria markedly change during cell cycles. Because subcellular sites and amounts of energy required for cells significantly differ during the cell cycle and maturation stages, intracellular localization and mitochondrial functions should also change depending on their status. In fact, Lee et al. [42] showed that the microtubular association of mitochondria was apparent in HeLa cells that grew rapidly, particularly at interphase of the cell cycle, while it disappeared during their mitotic phase. Thus, mitochondrial traffic changes markedly depending on the cell cycle.
Information about the structure, function, and intracellular traffic of mitochondria in mammalian oocytes is highly limited as compared with that of somatic cells. Changes in intracellular distribution of mitochondria and its relationship with the cytoskeleton during the meiotic maturation of oocytes have been reported in some mammals [43]. The GV stage of murine oocytes shows characteristic localization of mitochondria in the perinuclear region. Because the perinuclear accumulation of mitochondria is associated with the formation of a microtubule organizing center, this traffic has been postulated to depend on the microtubular network [44].
Calarco [45] showed that depolymerization of microfilaments by cytochalasin B failed to inhibit the perinuclear localization of mitochondria. In contrast, mitochondria preferentially localized in the periplasmic region of porcine GV oocytes while they moved to the central region of cells during GVBD and anaphase I. Mitochondria remained localized preferentially around the central region of mature oocytes. Because the presence of specific inhibitors of microtubules, but not of microfilaments, blocked mitochondrial movement, this translocation has been postulated to depend on the network of microtubules [46]. Similar changes in the localization of mitochondria during meiosis have also been observed with human oocytes through a mechanism that could be inhibited by colchicine but not by cytochalasin B [47]. However, Yu et al. [48] reported that a network of cortical microfilaments also underlies the mechanism of mitochondrial localization in murine oocytes. Furthermore, Duan et al. [49] showed that Rho-associated coiled coil-forming kinase played a role in the preferential distribution of mitochondria around the spindle of murine MI oocytes. Recent studies in our laboratory also showed that microfilaments rather than microtubules played a critical role in the vectorial transport of mitochondria in porcine GV oocytes [50].
Fluorescence-labeled oocyte mitochondria injected into the central region of donor oocytes preferentially moved to the subcortical region close to plasma membranes, whereas those injected into the subcortical region dispersed along plasma membranes. These mitochondrial vectorial movements were inhibited by specific microfilament inhibitors (such as cytochalasin B and D) but not by microtubule inhibitors (such as colcemid and nocodazole). These observations suggest that both microtubules and microfilaments underlie the mechanism of mitochondrial traffic in mammalian oocytes, though their dependency on the two cytoskeletons differs based on the species and/or the maturation stages of cells.
Although the possible involvement of motor protein families in the mechanism of mitochondrial traffic has also been suggested with mammalian oocytes, detailed information is lacking. Microfilament-associated mitochondrial traffic in neurons has been shown to be driven by the myosin motor protein family [33, 51, 52]; myosin transports various organelles from the minus end to the plus end of microfilaments. In cultured chicken neurons, myosin V has been shown to be associated with the movement of mitochondria in vitro at similar rates to those of axonal transport on microfilaments [34]. Thus, myosin V seems to play a major role in the actin-dependent mitochondrial traffic in axons [53, 54].
Dalton and Carroll [55] reported that preferential localization of mitochondria around the spindle of murine MI oocytes was suppressed by inhibiting dynein and accelerated by inhibiting kinesin. These observations suggest that both kinesin and dynein also play important roles in the regulation of mitochondrial traffic in mammalian oocytes. Possible roles for myosins in the regulation of mitochondrial traffic in mammalian oocytes remain unknown, and this requires further study.
Energy Metabolism in Mammalian Oocytes
A variety of physicochemical events occurring in actively metabolizing cells require large amounts of ATP synthesized by glycolysis in the cytoplasm and/or oxidative phosphorylation in the mitochondria. The former pathway depends on glucose uptake across plasma membranes, whereas the latter depends on the cellular availability of pyruvate and lactate, which are precursor substrates for acetyl-CoA used for the TCA cycle. Cellular uptake of glucose principally depends on the presence of Na+-dependent and independent glucose transporters.
Fertilization of mammalian oocytes requires a wide variety of cellular events including their maturation from the GV stage to MII; this is associated with the accumulation of cortical granules at the subcortical region and formation of plasma membrane lipid rafts. Furthermore, fertilization induces striking changes in oocytes such as excretion of cortical granules, Ca++-oscillation to inhibit polyspermy, chromosomal rearrangement followed by spindle formation, polar body extrusion, cell cleavage, and formation of blastocysts. All these events require large amounts of energy. Hence, cellular ATP should be supplied in sufficient quantities through glycolysis and/or oxidative phosphorylation in mitochondria. In fact, energy metabolism has been suggested to play important roles in the maturation of human, murine, and bovine oocytes [18, 19, 56–60]. However, despite the presence of glucose transporter 1, 3, and 8 (SLC2A1, SLC2A3, and SLC2A8) in bovine, human, sheep, and rhesus monkey oocytes [61–64], their activity to uptake and use glucose is fairly low, presumably due to low activity of phosphofructokinase, a rate-limiting enzyme in glycolysis [65–67].
It should be noted that maturing oocytes, particularly those in the ovary (and just after ovulation), are surrounded by nursing granulosa cells that express SLC2A4, a functional high-affinity glucose transporter [68–70]; the rate of glucose uptake by SLC2A4 depends on the presence of insulin and/or insulin-like growth factors but not on extracellular glucose concentrations [71]. Tompson et al. [72] reported that cumulus cells metabolize glucose at a rate 23 times higher than that of oocytes, whereas the former cells consume oxygen at a rate of 3.2 times greater than the latter. Furthermore, even in the absence of glucose, oocytes denuded of their nursing cumulus cells underwent maturation in the presence of pyruvate [73]. Based on such observations, the surrounding cumulus cells have been postulated to supply pyruvate to oocytes as a substrate for mitochondrial electron transport.
The structure and function of mitochondria differ significantly depending on cell types and stages of cell differentiation. In fact, the structure of mitochondria in oocytes is characterized by their round shape with minimum amounts of cristae. Ovarian oocytes in newborn babies are arrested after their birth at prophase of the first meiotic division; then, on a daily basis, some are selected and gradually increase their cell size. Unlike in most somatic tissue stem cells, the mitochondria in oogonial stem cells show characteristic properties of being enriched with cristae that are normally observed in actively functioning somatic cells during maturation and differentiation. The cristae-like structure is not apparent with mitochondria observed in immature mammalian oocytes [59, 74–76]. This round shape of oocyte mitochondria is preserved even after their cleavage up to the 8–16 cell stage in cattle [75] and to the morula stage in human [75].
The relationship between cellular ATP levels and developmental competence of oocytes was studied in IVF procedure [18, 19, 56–60]. Assuming that most oxygen was utilized for oxidative phosphorylation during the pre-compaction stages of bovine oocytes, approximately 96% of cellular ATP would have been synthesized by mitochondria [77]. However, mitochondria in immature bovine oocytes utilized approximately 63% of oxygen consumed, whereas those in mature cells used only 43% [78]. Mitochondria in murine embryos consumed approximately 30% of oxygen [79]. Thus, the contribution of mitochondria to ATP synthesis in oocytes seems to differ with maturation stages and from one species to another. It should be noted that cellular ATP levels are appropriately regulated by a dynamic equilibrium between ATP synthesis and utilization by glycolysis and/or oxidative phosphorylation. Because mammalian oocytes have exceptionally large numbers of mitochondria (20,000–100,000/cell) [22–24], they seem to have sufficient capacity to synthesize the necessary amounts of ATP and maintain its steady-state level required for cell maturation, fertilization, and blastocyst formation despite the minimum enrichment of cristae-like structures in the mitochondria. Thus, it is not surprising that ATP levels remained unchanged during the maturation of oocytes. In fact, van Blerkom et al. [18] reported that ATP levels in murine oocytes remained unchanged during their maturation. In contrast, Brevini et al. [80] and Iwata et al. [60] reported that ATP levels changed significantly during the maturation of oocytes from pigs and cattle. Thus, the bioenergetic properties of maturing oocytes require further study.
Genetic and Epigenetic Control of Mitochondria
Mitochondria have their own DNA (mtDNA) and play important roles in a wide variety of cellular metabolism including ATP synthesis, maintenance of membrane potentials, induction of apoptosis, and regulation of aging. Subcellular localization of mitochondria and their activity to synthesize ATP differs significantly from one cell type to another depending on their role in supporting the survival of organisms. Thus, the cell-specific properties of mitochondria should be regulated appropriately by nuclear and/or mitochondrial genes. It should be noted that nuclear genes are principally responsible for the transcription of mRNAs followed by synthesis of the proteins required by mitochondria, whereas mtDNA is predominantly responsible for the synthesis of rRNAs and a small number of proteins in electron transport chains [81]. Accumulating evidence suggests that mitochondrial dysfunction and various diseases cause epigenetic modification of nuclear DNA (nDNA) [82–84].
Epigenetics is an important mechanism that regulates gene expression without changing the sequence of genomic DNA either in a permanent or transient manner [85]. Epigenetic modification of nDNA involves at least three systems including DNA methylation (5-mC) or hydroxymethylation (5-hmC) at the position of carbon five in cytosine residues juxtaposed to a guanine base (termed CpG dinucleotides), covalent modification of the N-terminal tails of histones (two H3, H4, H2A, and H2B), and non-coding RNA-associated regulation of gene expression [86–93]. These systems are currently considered to initiate and sustain epigenetic cell changes [94].
An early study conducted three decades ago reported that there was no methylation of mtDNA [95]. Subsequently, the presence of low levels of methylation restricted to CpG dinucleotides in mtDNA was reported with several species [96–98]. Methylation of CpG in mammalian mtDNA has been shown to suppress gene expression at similar levels to those of nDNA [99]. This observation suggests that 5-mC is susceptible to mutation in mtDNA, and its modification is important for the regulation of mitochondrial function. It has been demonstrated that murine embryonic cells have nDNA methyltransferase 1 (DNMT1), which is transferred to the mitochondrial matrix to modify mtDNA [83]. The enzyme translocation is regulated by the conserved mitochondrial-targeting sequence located at the upstream region of the transcription start site within the nuclear gene. These findings suggest the possibility that the epigenetic regulation of mtDNA by DNMT1 also occurs in mitochondria.
The mitochondrial genome in mammals encodes 13 proteins that constitute the respiratory chain complexes; two rRNAs and 22 tRNAs specific to this organelle. All other proteins in mitochondria, including those necessary for mtDNA replication and transcription, are encoded in the nDNA. The proteins synthesized in the cytoplasm are transferred into mitochondria via fully specialized import systems, some of which recognize N-terminal mitochondrial-targeting sequences [100]. Unlike nDNA, mtDNA is not associated with histones. However, mtDNA is associated with protein-containing nucleoids [101].
Transcription of mtDNA depends on nuclear-encoded gene products [102]. Oxidative stress has been shown to stabilize peroxisome proliferator-activated receptor γ-coactivator 1α (PGC1α), which activates the transcription of several nuclear-encoded transcription factors including nuclear respiratory factor 1 (NRF1). PGC1α and NRF1 form a complex that up-regulates transcription of transcription factor of activated mitochondria (TFAM) and multiple members of the mitochondrial respiratory chain complexes [102]. Several nuclear-encoded genes involved in mitochondrial function are regulated by DNA methylation. Inversely, it has been shown that mitochondria regulate epigenetic modification in the nucleus. This observation suggests that mitochondria affect the level of cytosine methylation in nDNA through changing the flux of one-carbon units for the generation of S-adenosylmethionine (SAM), a donor of the methyl group for DNA methylation [82]. DNA methyltransferase catalyzes the methylation of cytosine residues at their carbon five positions by translocating the methyl group of SAM-CH3 [103–105].
Mitochondrial dysfunction might affect cellular production of SAM-CH3 and cause perturbation of the methylation of nDNA via cross talk between mitochondrial and nuclear genomes [106, 107]. Aberrant methylation of DNA at certain loci in the nuclear genome was associated with the deletion of mtDNA [82]. The study using mtDNA-depleted cells (ρ0 cells) suggested that depletion of mtDNA resulted in the aberrant methylation of promoter CpG islands (high CG-rich regions). The 5′ UTR comprising a CpG island in genetically modified ρ0 cells (143B ρ0) was found to be hypomethylated as compared with that in the parental cells that was completely hypermethylated at this region [82]. The authors concluded that partial loss of genomic DNA methylation was associated with the loss of mtDNA and/or mitochondria [82]. Replenishment of mtDNA deficient cells with wild-type mitochondria partially restored the methylation profiles similar to their original state. This observation suggested that mitochondria controls nDNA methylation.
Cross talk between mitochondrial and nuclear genomes has been suggested to play important roles in the mechanism of aging and carcinogenesis in which DNMT1 activity was perturbed [108, 109]. Mitochondrial dysfunction has been shown to participate in the occurrence of certain types of cancers [82, 110, 111] and neurodegenerative disorders [112]. Accumulating evidence suggests that mitochondrial dysfunction and a variety of diseases cause epigenetic modification of nDNA. This possibility requires further study.
Future Perspective
Aging-associated decrease in the quality and developmental competence of oocytes and increase in chromosomal abnormality of newborns are critical problems in human reproduction. A similar decrease in the quality and developmental competence of oocytes is also observed in other mammals. In fact, spontaneous aging and repeated ovulation are known to decrease the number and quality of ovulated oocytes in rodents. Sato et al. [113] reported that oxidative stress plays important roles in the mechanism of LH surge-induced ovulation in mice and rats. Kinetic analysis revealed that the superoxide radical generated by NADPH oxidase in and around granulosa cells in the ovary induced their apoptosis, thereby allowing ovulation of matured oocytes into the peritoneal cavity by a mechanism that could be inhibited by superoxide dismutase (SOD) [114]. Miyamoto et al. [4] reported that superoxide generated by mitochondria selectively induced the apoptosis of maturing oocytes, thereby regulating the number of oocytes that finally undergo ovulation. These observations suggest that oxidative stress underlies the mechanism of both ovulation and aging of the ovary, and this decreases the quality of oocytes remaining in this organ. Kinetic analysis revealed that repeated ovulation increased the number of aggregated mitochondria in oocytes and decreased their developmental competence in mice [4]. Repeated ovulation caused perturbation of subcellular distribution of mitochondria and gene expression of NRF1 and TFAM.
It has been well documented that oxidative stress enhances the mutation of genes both in nuclei and mitochondria; the rate of mutation is 10 times faster with the latter than with the former [114]. In fact, mutation and deletion of mtDNA in somatic tissues accumulated fairly rapidly with aging as compared with those of nDNA [115–118]. Mutation and/or deletion of mtDNA decreased cellular ATP levels presumably due to perturbation of the mitochondrial electron transport system [119]. Obesity, insulin resistance, diabetes, and maternal aging have been shown to change the structure, distribution, membrane potential of mitochondria, and mtDNA abundance with a concomitant decrease in the developmental competence of oocytes [120–123]. Mitochondrial dysfunction may decrease the fertility and induce the developmental arrest and retardation of human embryos [124–126]. Because mitochondria in embryos are inherited exclusively from oocytes [127], mutation and/or deletion of mtDNA in oocytes and embryos may underlie the etiology of mitochondrial diseases [128–132].
To maintain and improve mitochondrial function in oocytes, various nutrients with antioxidant nature have been tested. L-Carnitine has been shown to decrease oxidative injury of mitochondria, cells, and tissues both in vitro and in vivo [3, 133]. Administration of L-carnitine successfully inhibited oxidative stress in various cells and tissues and decreased tissue injury in animals that had received anticancer agents [134]. L-Carnitine also suppressed the aging of senescence-accelerated mice and mice with amyotrophic lateral sclerosis (ALS) [3, 135]. Furthermore, Hino et al. [136] reported that oral administration of L-carnitine successfully inhibited hypoglycemia-induced brain damage in rats. These observations suggest that L-carnitine has beneficial effects in suppressing the oxidative injury of mitochondria, cells, and tissues in vivo. Thus, we have hypothesized that L-carnitine may also suppress the aging-enhanced pathologic events in ovarian cells and tissues. In fact, oral administration of L-carnitine successfully suppressed the pathological events induced by repeated ovulation and by natural aging in mice, such as aggregation of mitochondria and a decrease in the developmental competence of oocytes [4]. Furthermore, L-carnitine added to a culture medium alleviated abnormal distribution, decreased membrane potential of mitochondria, and normalized the spindle structure after in vitro maturation following vitrification [137].
Dichloroacetic acid, an inhibitor of pyruvate dehydrogenase kinase, added to the culture medium increased mitochondrial membrane potential and decreased oxidative stress of oocytes in aged mice, thereby enhancing their developmental competence in vitro [138]. Coenzyme Q10 added to a maturation medium also suppressed the abnormal distribution and decreased the membrane potential of mitochondria in bovine oocytes and improved their mtDNA expression and developmental competence [139]. Thus, the similar effects of L-carnitine and related compounds that protect mitochondrial functions to improve the quality and developmental competence of oocytes should be tested further with human oocytes, both in vivo and in vitro.
Another approach to improve mitochondrial function and the developmental competence of oocytes has been tested by directly microinjecting ooplasm and/or mitochondria into recipient oocytes. In this context, ooplasm has been transferred from healthy donor cells to recipient oocytes obtained from patients undergoing infertility treatment who showed recurrent implantation failure presumably due to poor embryonic development. Approximately, 30 healthy babies were born after injection of donor ooplasm derived from fresh MII oocytes, frozen-thawed MII oocytes, and 3-PN zygotes [140–147]. Furthermore, transfer of ooplasm using an electrofusion method was found to improve the developmental ability of pig and bovine oocytes [148, 149]. These results suggest that the transfer of cytoplasmic factors seems to confer beneficial effects on oocytes having poor developmental competence. It should be noted that mammalian ooplasm contains a variety of cytoplasmic factors such as mRNAs, proteins, and various organelles including mitochondria. Hence, factors other than the mitochondria may have produced the beneficial effects on maturation and development of oocytes with poor developmental competence.
Nuclear transfer could replace most ooplasmic components other than nuclear constituents. Thus, the effect of nuclear transfer has been studied to evaluate the efficacy of ooplasm to support oocytes without causing possible inheritance of injured or mutated mtDNA that otherwise induces mitochondrial diseases. In fact, nuclear transfer has been shown to prevent the transmission of mtDNA from donor oocytes to the reconstructed embryos, embryonic stem cells, and offspring in mice, humans, and other primates [150–153]. Thus, nuclear transfer may be a useful method to avoid the possible inheritance of mitochondrial diseases. Nuclear transfer has also been tested to rescue the genetic material of oocytes with low developmental competence.
Takeuchi et al. [20] reported that GVs obtained from oocytes with low maturation and developmental competence, and photo-oxidatively damaged mitochondria, could be rescued by nuclear transfer into an intact ooplasm. Similarly, the developmental competence of MII chromosomes from oocytes challenged with in vitro aging was also rescued by transferring them into fresh MII ooplasm [154]. In contrast, Cui et al. [155] reported that the transfer of GVs from aged mouse oocytes into ooplasm from young animals failed to rescue aging-associated chromosomal misalignment during meiosis. Thus, the clinical impact of nuclear transfer to rescue oocytes with poor quality ooplasm requires further study.
The effects of the transfer of mitochondria to improve developmental competence of oocytes and embryos have also been studied. El Shourbagy et al. [156] reported that the transfer of mitochondria from good quality oocytes improved the fertilization rate of poor quality cells. Transfer of mitochondria isolated from granulosa cells inhibited the apoptosis of murine MII oocytes [157], whereas it improved the preimplantation development of poor quality bovine oocytes [158]. Similarly, Yi et al. [159] reported that the microinjection of liver mitochondria into 2-PN zygotes derived from both young and aged mice improved their preimplantation development. Furthermore, Tzeng et al. [160] reported that a successful pregnancy was achieved after transfer of mitochondria derived from granulosa cells to autologous oocytes from a patient with recurrent implantation failure. In contrast, Takeda et al. [161] reported that the microinjection of mitochondria obtained from cumulus cells suppressed the cleavage and development of oocytes. The reason for such discrepancies remains unclear.
It should be noted that the quality of the isolated mitochondria and/or donor cells used for the transfer would have affected these results. Furthermore, the properties and functions of mitochondria differ significantly among cells and tissues. In this context, typical mitochondria observed in healthy oocytes have a round shape with small amounts of cristae as described previously. Although the structure of mitochondria observed in most somatic cells changes dynamically, they generally have an elongated shape enriched with cristae (see Fig. 4.2). These structural and functional differences of donor mitochondria may affect the outcome of mitochondrial transfer into recipient oocytes. Evaluation of a suitable source(s) and the properties of donor cells seem to be critical for improving the competence of oocytes by using mitochondrial transfer. Mitochondria in embryonic stem cells and induced pluripotent stem cells generally have a round shape with few cristae as observed with oocytes [162–164].


Fig. 4.2
A mitochondrion in a somatic cell. The mitochondrion has an elongated structure. Magnification × 50,000; scale bar = 0.5 μm
Transfer of either ooplasm or nuclei to improve the competence of poor quality oocytes requires healthy donor cells. Furthermore, transfer of heterologous ooplasm and/or mitochondria may result in the occurrence of heteroplasmy [146, 147]. Nuclear transfer would cause perturbation of the cross talk between mtDNA and nDNA [150]. Autologous transfer of mitochondria obtained from somatic cells would avoid unfavorable interaction of the two genomes in mtDNA and nDNA. Mitochondrial transfer would be effective in the elimination of pathologic events arising from endogenous mitochondria. Further studies on effective methods are necessary to increase the maturation and developmental competence of oocytes from patients with infertility caused by diabetes, mitochondrial disease, and aging.
We hypothesize that mitochondria from oogonial stem cells could be potential donor specimens for transfer into recipient oocytes. Clinical trials using donor mitochondria from oogonial stem cells from the ovaries of IVF patients for transfer into autologous oocytes with poor developmental competence are currently under investigation.
Conclusion
Mitochondria play an important role not only as cellular powerhouse, but also as potential sources for reactive oxygen species that impair a wide variety of biomolecules, thereby inducing cellular aging and apoptosis. Cytoplasmic factors including mitochondria have been known to regulate the expression of nuclear genes to induce physiological maturation of oocytes. Quality of cytoplasm and mitochondria in oocytes could be improved practically by dietary intake of foods and some supplements that work as scavengers against reactive oxygen species. Such scavengers have been used successfully to maintain or improve the quality of oocytes during IVF. In vitro handling of cytoplasmic factors including mitochondria could be performed safely without disturbing cell integrity. Hence, possible effect of mitochondrial transfer to improve the clinical outcome of in vitro maturation procedure needs to be evaluated carefully.
References
1.
Faddy MJ, Gosden RG, Gougeon A, Richardson SJ, Nelson JF. Accelerated disappearance of ovarian follicles in mid-life: implications for forecasting menopause. Hum Reprod. 1992;7:1342–6.PubMed
2.
Tessa L, John R. A. Oxidative stress and ageing of the post-ovulatory oocyte. Reproduction 2013;146:217–27.
3.
Inoue M, Sato E, Nishikawa M, et al. Free radical theory of apoptosis and metamorphosis. Redox Rep. 2004;9(5):237–47. doi:10.1179/135100004225006010.PubMed
4.
Miyamoto K, Sato E, Kasahara E, et al. Effect of oxidative stress during repeated ovulation on the structure and functions of the ovary, oocytes, and their mitochondria. Free Radic Biol Med. 2010;49(4):674–81. doi:10.1016/j.freeradbiomed.2010.05.025.PubMed
5.
Liu N, Wu YG, Lan GC, Sui HS, Ge L, Wang JZ, Liu Y, Qiao TW, Tan JH. Pyruvate prevents aging of mouse oocytes. Reproduction. 2009;138(2):223–34. doi:10.1530/REP-09-0122 Epub 2009 May 22.PubMed
6.
Pincus G, Enzmann EV. The comparative behavior of mammalian eggs in vivo and in vitro: I. The activation of ovarian eggs. J Exp Med. 1935;62:655–75.
7.
Edwards R. Maturation in vitro of mouse, sheep, cow, pig, rhesus monkey and human ovarian oocytes. Nature. 1965;20:349–51.
8.
Edwards R, Bavister B, Steptoe P. Early stages of fertilization in vitro of human oocytes matured in vitro. Nature. 1969;221:632–5.PubMed
9.
Veeck LL, Wortham JW Jr, Witmyer J, et al. Maturation and fertilization of morphologically immature human oocytes in a program of in vitro fertilization. Fertil Steril. 1983;39:594–602.PubMed
10.
Cha KY, Koo JJ, Ko JJ, et al. Pregnancy after in vitro fertilization of human follicular oocytes collected from nonstimulated cycles, their culture in vitro and their transfer in a donor oocyte program. Fertil Steril. 1991;55:109–13.PubMed
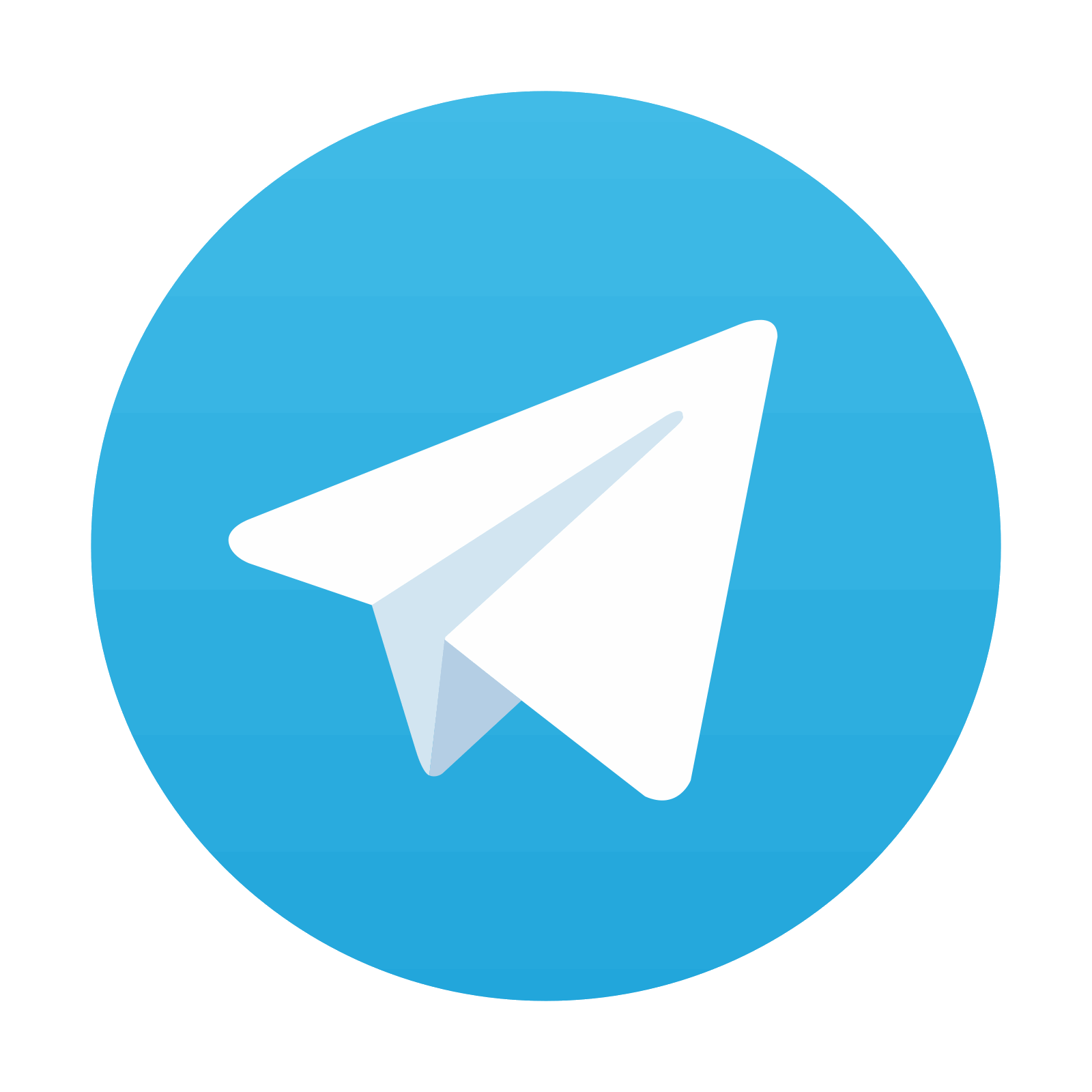
Stay updated, free articles. Join our Telegram channel
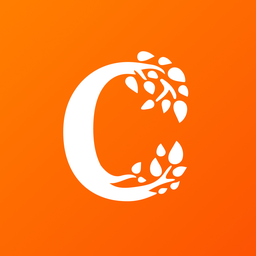
Full access? Get Clinical Tree
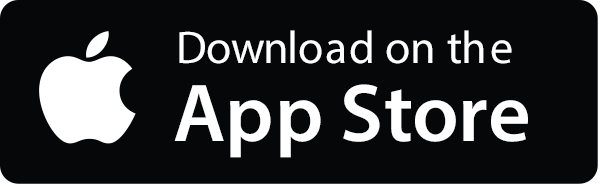
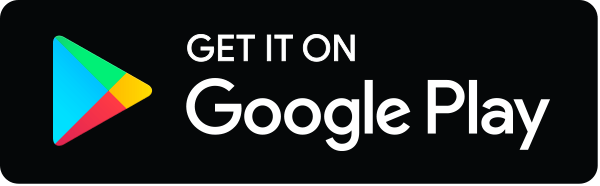