Method
Measurement
Advantages
Disadvantages
Value in drug development decisions
Fasting serum insulin and glucose; mathematic models include HOMA-IRa and QUICKIb
Venous serum insulin and plasma glucose are measured in blood samples drawn after an 8–12 h overnight fast
Insulin sensitivity (%S)
Technically simple; relatively inexpensive; provides an indication of insulin sensitivity in relation to hepatic glucose metabolism
Indirect assessment of insulin action; only assesses metabolism in basal (non-stimulated) state; insulin resistance may be underestimated in the presence of hyperglycaemia
May provide useful exploratory data in early-phase studies; results should be confirmed with a dynamic test
Frequently sampled intravenous glucose tolerance test (FSIVGTT)
Glucose, insulin and C-peptide responses to an intravenous bolus of glucose; minimal model analysis of
Minimal model yields insulin-sensitivity index (SI) and glucose effectiveness (SG)
Provides dynamic data; widely used in clinical metabolic research
Indirect integrated assessment of glucose metabolism; questionable relevance to normal physiology
Limited value
Mixed-meal tolerance test (MMTT)
Plasma glucose and serum insulin responses at defined intervals to a standardized meal
Area under the curve (AUC) for insulin; mathematical models of insulin and glucose responses (e.g., Matsuda index; Stumvoll index)
Provides data relevant to normal physiology; flexible, i.e. nutrient components can be adjusted; assesses integrity of incretin axis
Indirect assessment of insulin action; issues of intra-individual and between-individual variability; affected by gastric emptying rate
May be of value in providing early signal of effects of drugs on insulin action
Oral glucose tolerance test (OGTT)
Glucose, insulin and/or C-peptide responses to 75 g oral glucose
AUC for insulin; mathematical models of insulin and glucose responses (e.g. Matsuda index; Stumvoll index
Simple; reference methods for diagnosing diabetes and impaired glucose tolerance; vast scientific literature
Indirect assessment of insulin action; intra-individual and between-individual variability; β-cell response to secretagogues other than glucose not assessed; affected by gastric emptying rate
May be of value in providing early signal of effects of drugs on insulin action
Insulin tolerance test (ITT)
Response of blood glucose to an intravenous bolus of glucose
Glucose disposal rate (KITT)
Technically simple
Risk of hypoglycaemia; cannot partition between insulin-mediated glucose disposal and suppression of hepatic glucose production
Limited role in diabetes drug development
Open-Loop Methods
Method | Measurement | Advantages | Disadvantages | Value in drug development decisions | |
---|---|---|---|---|---|
Insulin suppression test (IST) | Somatostatin is infused to suppress endogenous insulin secretion; insulin and glucose are infused to achieve steady-state plasma glucose | Steady-state plasma glucose (SSPG) | Reproducible steady-state method which eliminates endogenous insulin secretion and assesses insulin-mediated glucose disposal | Indirect assessment of insulin action on glucose metabolism; labour-intensive; relatively inflexible; hepatic insulin sensitivity cannot be determined | Limited value |
Insulin-sensitivity clamp (two-step hyperinsulinaemic-euglycaemic clamp) | Insulin is infused to provide steady-state hyperinsulinaemia at predetermined insulin concentration; variable rate glucose is infused to maintain plasma glucose at euglycaemia | Glucose disposal rate (M); M/I; insulin-sensitivity index (SIclamp) | Direct measure of insulin-mediated glucose disposal; reproducible; low coefficient of variation; may be readily combined with complementary techniques, e.g. isotopic determination of glucose turnover, indirect calorimetry; automatic clamps using the Biostator offer advantages over manual clamps | Labour intensive; requires skilled technical staff | Generally regarded as the reference method for determining insulin sensitivity |
Determination of Pharmacodynamic Properties of Insulin Formulations
Method | Measurement | Advantages | Disadvantages | Value in drug development decisions | |
---|---|---|---|---|---|
Time-action profile – glucose clamp (euglycaemic clamp) | Insulin is administered by subcutaneous injection/inhalation, etc.; hypertonic glucose is infused intravenously at a variable rate to maintain plasma glucose at euglycaemia | Maximal glucose infusion rate (GIRmax); time to GIRmax (t max); area under the curve (AUC0-T ) | Yields simultaneous detailed pharmacodynamic and pharmacokinetic data | Labour intensive; requires skilled technical staff; assessment of ultra-long acting insulins has limitations | Clamp-derived time-action profiles for insulin and biosimilar insulins are required by US and European regulators for market approval of new insulins |
Conclusions
Of the available methods for quantifying insulin action, the euglycaemic glucose clamp technique is widely regarded as the reference method. The hyperinsulinaemic-euglycaemic glucose clamp provides robust and reproducible measures of insulin sensitivity. When combined with isotopic determination of glucose turnover, the sensitivity of hepatic glucose metabolism to insulin can also be quantified. The glucose clamp technique is highly adaptable and may be complemented by methods such as indirect calorimetry to measure substrate oxidation or tissue biopsy of fat or muscle to examine enzyme activity in the stimulated state.
The euglycaemic/isoglycaemic glucose clamp technique is used extensively to assess the time-action profiles of insulin preparations. Glucose clamp studies are resource-intensive. All variants of the glucose clamp technique, including automated clamps, are to some extent operator dependent and require experienced technical staff to ensure accurate and reproducible data.
Introduction
Insulin is a pivotal hormone controlling critical energy functions including regulation of glucose and lipid metabolism at cellular, tissue, and whole-body level. Impaired action of insulin, i.e. insulin resistance, is a well-documented feature of many physiological and pathological states (Table 1.1) [1–4]. These range from temporary subclinical decrements in insulin action to rare genetic syndromes of severe insulin resistance requiring innovative pharmacotherapeutic approaches [5]. From a global public health perspective, excess adiposity is the most common form of acquired insulin resistance. Impaired insulin action is a major modifiable factor in the pathogenesis of glucose intolerance and type 2 diabetes and is closely associated with the adverse profile of risk factors for cardiovascular disease [6].
Table 1.1
Physiological and pathological states associated with whole-body insulin resistance
Physiological states |
Adolescence |
Pregnancy (2nd and 3rd trimesters) |
Luteal phase of the menstrual cycle |
Postmenopausea |
Ageinga |
Common pathological conditions |
Obesityb |
Glucose intolerance |
Type 2 diabetes |
Metabolic syndromec |
Sedentary lifestyle (vs. regular physical activity) |
Non-alcoholic fatty liver disease/steatohepatitis |
Insulin Physiology and Metabolic Regulation
Hepatocytes, skeletal myocytes, and white adipocytes are regarded as classic insulin-responsive tissues [7]. Most cells express surface insulin receptors. Insulin regulates glucose metabolism both through direct actions [8] and in part by influencing interorgan crosstalk pathways including the synthesis and secretion of fat-derived adipocytokines (Table 1.2) [9]. Insulin signalling in the brain influences energy balance and peripheral glucose metabolism [10]. Other nonclassic target tissues for insulin include the heart [11], skeleton [12], brown adipocytes [13], and ovaries [14]. The metabolic function of these organs may be favourably or unfavourably affected by insulin-sensitizing drugs [15]. The range of physiological actions of insulin has expanded beyond regulation of carbohydrates and other macronutrients to include antioxidant, anti-inflammatory, and vascular effects (Table 1.3) [16, 17].
Table 1.2
Classic metabolic actions of insulin
Direct effects | Indirect effects | |
---|---|---|
Skeletalmusclea | ↑Glucose uptake | ↓ NEFA availability and oxidation |
↑Glucose oxidation | ||
↑ Glycogen synthesis | ||
Liver | ↓ Glucose output | ↓ NEFA availability and oxidation |
↓ Glycogenolysis | ||
↓ Gluconeogenesis | ||
↑ Glycogen synthesis | ||
↑ Glycolysis | ||
↑ Lipogenesis | ||
Adiposetissue | ↑ Glucose uptake | ↓ Lipolysis |
↑ Lipogenesis | Regulation of adipocytokines |
Table 1.3
Nonclassic actions of insulin
Antioxidant |
↓ Reactive oxygen species |
Anti-inflammatory |
↓ NFκB ↓ C-reactive protein |
Antithrombotic |
↓ Tissue factor ↓ platelet activation |
Pro-fibrinolytic |
↓ Plasminogen activator inhibitor-1 |
Vasodilatory |
NO-mediated improvements in endothelial function |
Large vessel compliance |
Lipid regulation |
↓ Hepatic production of very-low-density lipoproteins |
Sympathetic nervous system |
↑ Activation |
Anti-atherosclerotic actions |
Apo E null mouse, IRS-1 null mouse |
Cellular Insulin Signalling
In peripheral tissues, e.g. muscle and fat, insulin must leave the intravascular compartment and traverse the interstitial space before interacting with cellular insulin receptors [18]. The insulin receptor is a transmembrane heterodimer comprising two α- and two β-subunits (Fig. 1.1). Binding to the α-subunit induces a conformational change resulting in release of the inhibitory effect of the α-subunit and autophosphorylation of tyrosine residues in the β-subunit [19]. Insulin receptor tyrosine kinase phosphorylates and recruits substrate adaptors such as the insulin receptor substrate (IRS) family of proteins. This initiates molecular events that result in translocation of the facilitative glucose transporter (GLUT4) from the cytosolic vesicles to the cell membrane [20, 21]. Fusion of GLUT4 with the cell membrane transports glucose into the cell where it is phosphorylated to glucose-6-phosphate. A post-binding cascade of phosphorylation/dephosphorylation reactions leads to activation of key enzymes including glycogen synthase and pyruvate dehydrogenase. In the presence of hyperinsulinaemia, glucose-6-phosphate is mainly (approximately 70 %) polymerized to form glycogen; the remainder enters the glycolytic pathway and is either oxidized or converted to lactate [22].
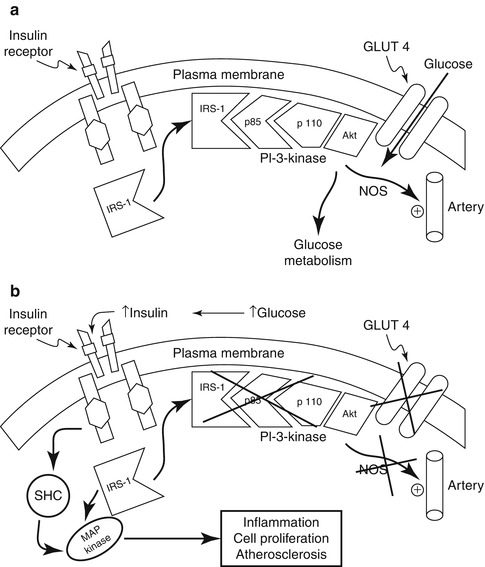
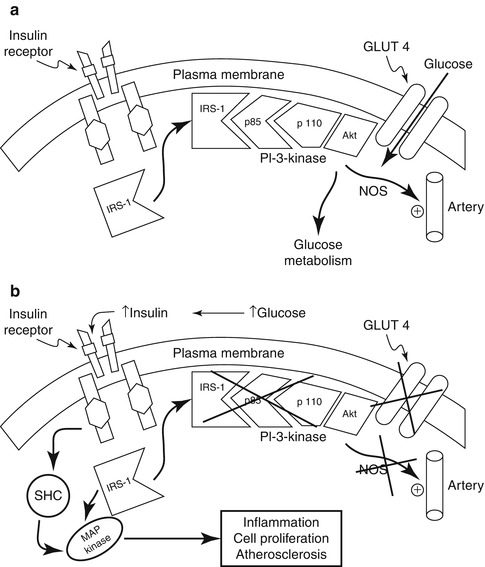
Fig. 1.1
(a) Insulin signal transduction system in individuals with normal glucose tolerance. NOS, nitric oxide synthase. (b) In patients with type 2 diabetes, insulin signalling is impaired at the level of insulin receptor substrate (IRS)-1 leading to decreased glucose transport/phosphorylation/metabolism and impaired nitric oxide synthase activation/endothelial function. At the same time, insulin signalling through the mitogen-activated protein (MAP) kinase pathway is normally sensitive to insulin. The compensatory hyperinsulinaemia due to insulin resistance in the IRS-1/phosphatidylinositol-3 (PI-3) kinase pathway results in excessive stimulation of the former pathway, which is involved in inflammation, cell proliferation, and atherogenesis. SHC Src homology collagen (With kind permission from Springer Science + Business Media: DeFronzo RA. 2010. [46])
Insulin Resistance
Insulin resistance may be defined as a state in which physiological concentrations of insulin produce a less than normal biological response [27]. The assessment of insulin sensitivity in clinical practice is recognized as being problematic. Accurate quantification of insulin action is possible only within a specialized clinical research setting. While obesity is closely associated with insulin resistance, body mass index (BMI) is a relatively unreliable indicator of whole-body insulin sensitivity (essentially the inverse of insulin resistance) [28]. Visceral adiposity, which is often accompanied by chronic low-grade systemic inflammation and alterations in cytokine physiology, is more closely associated with insulin resistance and risk factors for cardiometabolic diseases (see below) [29]. Clinically useful biomarkers for insulin resistance remain underdeveloped [30].
Cardiometabolic Consequences of Insulin Resistance
Insulin resistance is strongly implicated in the pathogenesis of hyperglycaemia, dyslipidaemia, and vascular disease [31–33]. It is estimated that approximately 30 % of the variability in insulin-mediated glucose uptake is accounted for by BMI, with factors such as physical activity levels and genetic influences contributing to the remainder of the variance [34]. Ectopic fat deposition, which may occur in the liver, skeletal muscle, the myocardium, and the pancreas, is associated with organ dysfunction and decreased insulin action both locally and more systemically [32, 35].
The leading cause of premature mortality in patients with diabetes is cardiovascular disease [36]. The metabolic syndrome (also known as the insulin resistance syndrome) encompasses the major obesity-associated clinical risk factors for atherothrombotic vascular disease, i.e. central adiposity, hyperglycaemia, dyslipidaemia (hypertriglyceridaemia or low levels of high-density lipoprotein cholesterol), and hypertension [37]. At the cellular molecular level, maintained insulin stimulation of the MAP kinase pathway by hyperinsulinaemia has been proposed as a pathway to atherosclerosis activation of inflammatory pathways (see Fig. 1.1) [38].
Insulin Resistance in the Pathogenesis of Type 2 Diabetes
Using quantitative techniques the majority of subjects with impaired glucose tolerance and patients with type 2 diabetes are insulin resistant, together with 25 % of otherwise apparently healthy normoglycaemic individuals [1, 39]. According to a widely accepted model, islet β-cells respond to reduced insulin signalling in target tissues with an appropriate increase in secretion of the hormone [39, 40]. If the rise in insulin production is less than the amount required for full metabolic compensation, glucose intolerance develops; progressive β-cell failure leads to degrees of glucose intolerance that may culminate in type 2 diabetes [39, 41].
In widely cited early study, Jerrold Olefsky, Orville Kolterman, and colleagues used the hyperinsulinaemic-euglycaemic glucose clamp technique to construct dose-response curves for insulin action across a range of insulin concentrations in subjects with obesity with or without type 2 diabetes. Defective insulin-mediated glucose disposal was most marked in the more insulin-resistant, hyperinsulinaemic obese subjects [42]. In less insulin-resistant nondiabetic obese individuals [42] and in subjects with impaired glucose tolerance [43], there was a rightward shift in the whole-body glucose disposal curve indicative of decreased insulin sensitivity. This was considered to be consistent with a defect in insulin action at the level of the insulin receptor (Fig. 1.2) [27, 42, 43]. In patients with type 2 diabetes, an additional defect was evident with an inability to achieve maximal response even in the presence of very high insulin concentrations (see Fig. 1.2) [42, 43]. The latter observation is characteristic of type 2 diabetes and is considered to be consistent with impaired insulin signalling distal to the interaction of the hormone with its receptor [43].
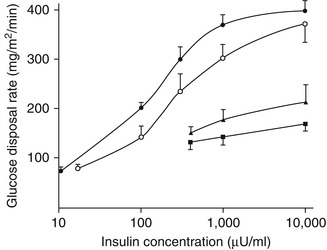
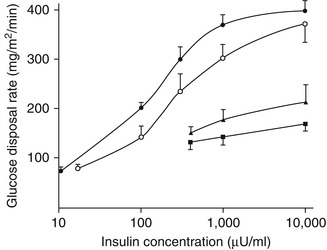
Fig. 1.2
Mean dose-response curves for control subjects (solid circles), subjects with impaired glucose tolerance (open circles), and nonobese (solid triangles) and obese (solid squares) subjects with type 2 diabetes. Hyperinsulinaemic-euglycaemic clamps were performed at multiple circulating insulin levels. The dose-response curve for the subjects with impaired glucose tolerance is shifted to the right relative to the health controls. This decrease in insulin sensitivity has been interpreted as consistent with a defect at the level of the insulin receptor. The subjects with type 2 diabetes show a more profound impairment of insulin-mediated glucose disposal. Not only is the shift to the right more pronounced, there is also a decrease in maximal insulin action, i.e. reduced insulin responsiveness. This is considered to be indicative of defects in the insulin signalling pathway distal to the interaction of insulin with its receptor (Data from Kolterman et al. [43] with permission) To convert μU/mL to pmol/L, multiply by 6.0
Post-binding intracellular defects in insulin action that have been described in type 2 diabetes include impaired transmembrane glucose transport, reduced glucose phosphorylation, lower rates of glucose oxidation, and reduced glycogen synthesis [22]. Defective insulin action, compounded by impaired physiological suppression of glucagon secretion [44], leads to fasting and postprandial hyperglycaemia [45]. In health, hepatic glucose production is appropriately suppressed by hyperinsulinaemia following a meal. Higher insulin levels are required to suppress glucose output by the liver in the presence of insulin resistance [43]. In subjects with type 2 diabetes, rates of postprandial glucose release into the circulation are increased. This glucose originates partly from endogenous sources, i.e. liver and kidney, due to a combination of increased glycogenolysis and gluconeogenesis, in concert with decreased splanchnic glucose uptake [45]. In absolute terms postprandial glucose disposal by skeletal muscle is not significantly impaired relative to nondiabetic subjects as a consequence of the mass action effect of hyperglycaemia [45]. However, the metabolic clearance rate of glucose, which takes prevailing hyperglycaemia into account, is reduced. In patients with type 2 diabetes, impaired insulin-mediated glucose disposal is associated with reduced non-oxidative metabolism, i.e. glycogen synthesis, in skeletal muscle [46].
Insulin Sensitivity: A Therapeutic Target in Type 2 Diabetes and Cardiovascular Disease
Improving insulin sensitivity through weight reduction and physical exercise remains the cornerstone of management of type 2 diabetes [47]. However, lifestyle modifications alone rarely provide adequate metabolic control and glucose-lowering medications are usually required [48, 49]. Insulin sensitizers are effective glucose-lowering agents [50]. The biguanide metformin, which lowers blood glucose primarily by improving hepatic insulin action, is widely regarded as the drug of choice [49, 51]. In the Diabetes Prevention Program, metformin reduced progression from glucose intolerance to type 2 diabetes in high-risk obese subjects [52]. Protection against the development of diabetes has also been reported with for thiazolidionediones [53].
Insulin-sensitizing drugs may be of value in preventing cardiovascular events [54, 55]. However, some investigators consider that this evidence supporting this assertion remains less than conclusive [56, 57]. The controversy surrounding the reported increase in myocardial infarction with rosiglitazone highlighted the potential for molecule-specific adverse effects even within a class of glucose-lowering agents [58, 59]. Safety concerns have led to discontinuation of the development other insulin-sensitizers including tesaglitazar [60] and muraglitazar in 2006 [61] and aleglitazar in 2013 [62]. Better understanding of peroxisome proliferator-activated receptor (PPAR-γ) signalling may help reduce or eliminate adverse effects of fluid retention, weight gain, congestive cardiac failure, and skeletal fractures [15, 63]. The identification of novel mechanisms of insulin resistance, e.g. adipose tissue inflammation [64], has opened the door to new therapeutic approaches [65].
Principles of Insulin Therapy
Exogenous insulin is used in the treatment of all patients with type 1 diabetes and in a substantial proportion of patients with type 2 diabetes [66]. In the latter population, insulin is usually deferred until other glucose-lowering strategies have proved insufficient [67]. Many technical advances in purity, pharmacokinetics, and delivery systems have been achieved since the introduction of insulin into clinical practice in 1922 (see below; Tables 1.4 and 1.5). Nonetheless, optimal insulin replacement remains a formidable therapeutic challenge. During the 1980s the advent of recombinant DNA technology permitted the creation of insulin analogues with improved pharmacokinetic (PK) properties [68]. This advance facilitated the use of so-called basal-bolus intensive insulin regimens that aim to reproduce the complex and finely tuned physiological pattern of insulin secretion [69–71]. Rapid-acting insulin analogues (e.g. insulin aspart, insulin lispro, insulin glulisine) [72] and analogues with prolonged action (e.g. insulin glargine, insulin detemir, insulin degludec) are now well established in clinical practice [73]. More recently, ultra-rapid-acting insulin formulations, injectable, e.g. hyaluronidase [74, 75], VIAject® [75, 76], and inhaled [77, 78], have been evaluated; in June 2014 the FDA approved the first inhaled human insulin (Afrezza®) since Exubera in 2006 [79]. The quest for a viable orally delivered insulin continues to drive clinical research activity [80]. In parallel, basal insulin analogues with ultra-long durations of action with potential for once-weekly administration are being developed [81].
Table 1.4
Chronological evolution of insulin therapy
Animal insulin |
Beef |
Pork |
Highly purified insulins |
Monocomponent insulin |
Human insulin |
Recombinant DNA technology |
High-strength insulin |
U500 human insulin |
U300 insulin glargine |
Insulin analogues |
Rapid acting |
Long acting |
Ultra-long acting |
Inhaled insulin |
Exubera® |
Afrezza® |
Biosimilar insulins |
‘Smart’ insulins, i.e. glucose-responsive |
Premixed combinations with glucagon-like peptide-1 agonists, e.g., Ideg Lira (insulin degludec + liraglutide) |
Table 1.5
Modified insulin preparations with altered pharmacokinetics
Isophane |
Neutral protamine hagedorn |
Lente |
Semi-lente |
Lente |
Ultralente |
Protamine zinc insulin |
Modified analogue (retarded action) |
Pegylatation |
Fc- carrier |
Absorption enhancers (accelerated action) |
Hyaluronidase |
EDTAa destabilization of insulin hexamers |
Type 1 Diabetes
Modern intensive insulin regimens aim to sustain near-normal blood-glucose profiles, including low postprandial glycaemic excursions [70]. On a day-to-day basis, the risk of acute metabolic decompensation, i.e. hyperglycaemia and diabetic ketoacidosis, resulting from insufficient insulin must be balanced against the risk of hypoglycaemia. The latter, which is the main limiting factor to achieving glycaemic goals, occurs when insulin action is in excess of physiological requirements. The quest for insulin preparations and regimens that further improve this risk-benefit equation remains a research priority. Much effort continues to be directed towards the creation of a closed-loop ‘artificial pancreas’ [82]. Glucose-responsive insulins that aim to match insulin availability to ambient glucose levels are also under investigation [83]. Pramlintide, glucagon-like peptide (GLP)-1 agonists, dipeptidyl peptidase (DPP)-4 inhibitors, and leptin have been explored as adjunctive therapies.
Type 2 Diabetes
In patients with type 2 diabetes other glucose-lowering agents are often used in combination with insulin with the aim of attenuating unwanted aspects of insulin therapy [84, 85]. The risk of severe hypoglycaemic events is generally lower than in patients with type 1 diabetes [86]. This risk increases over time as β-cell function progressively declines and physiological counterregulatory responses to hypoglycaemia become impaired [86]. Weight gain is a frequent and unwanted effect of insulin therapy. With greater degrees of obesity, daily insulin requirements may become cumbersome. Use of high concentration insulin preparations reduces the required volume of insulin [87]. While U500 insulin is unmodified soluble human insulin, its pharmacokinetics resemble those of an intermediate-duration insulin [88].
Measurement of Insulin Action in Humans
In clinical studies during the 1930s using an oral glucose challenge with a concomitant injection of insulin, Professor Sir Harold Himsworth of London University made a series of classic scientific observations that continue to inform current views of quantifying insulin action [89]. Himsworth divided diabetes into insulin-sensitive and insulin-insensitive subtypes, effectively pre-empting the modern diagnostic categories of type 1 and type 2 diabetes [90].
A major step forward came several decades later with the development of a method to measure insulin in the circulation [91], an achievement for which Rosalyn Yalow and Solomon Berson were awarded the Nobel Prize in medicine. It rapidly became clear that obesity and glucose intolerance were conditions characterized by elevated systemic insulin concentrations [92]. This led John Karam and Gerold Grodsky to speculate about antagonism of insulin action from an excess of nonesterified fatty-acid levels in muscle. By this time Professor Sir Philip Randle and colleagues at the University of Cambridge had described the glucose-fatty-acid cycle in rat myocardial and skeletal myocytes [93]. Kenneth Zierler and David Rabinowitz examined the dose-response effects of intrabrachial insulin infusion on glucose, fatty acids, and potassium metabolism in elegant isolated forearm experiments [94].
During the 1970s techniques for quantifying insulin action at the whole-body level, most notably the hyperinsulinaemic euglycaemic glucose clamp, were devised and implemented (see below) [95]. The 1980s was a decade of many notable advances concerning insulin receptor binding and intracellular signal transduction [96, 97]. Using techniques such as indirect calorimetry, isotopic tracers, and tissue biopsy, the details of defective insulin action in obesity, diabetes, and related cardiometabolic disorders became ever clearer [2].
The 1990s saw the introduction of the thiazolidinediones, a therapeutic advance that was later to have a profound and enduring impact on safety requirements for glucose-lowering agents [58, 98, 99]. In the 1980s and 1990s, the glucose clamp technique was also used to systematically evaluate time-action profiles of novel insulin formulations [100].
Classification of Methods for Measuring Insulin Sensitivity
Methods in current use for quantifying insulin action may be classified according to whether metabolism is studied at levels of insulin relevant to normal physiology or at pharmacological concentrations of the hormone. An alternative classification considers whether the feedback loop between the β-cells and insulin-sensitive tissues remains intact or is interrupted by pharmacological intervention (Table 1.6) [4]. Methods may also be subdivided into either whether they are basal or dynamic tests or whether they involve an experimental steady state of hyperinsulinaemia.
Table 1.6
Investigative techniques for the assessment of insulin action in man
Closed-loop assessment of basal metabolism |
Fasting insulin |
Homeostasis model assessment (HOMA) |
Closed-loop dynamic tests |
(a) Endogenous insulin |
Oral glucose tolerance test, e.g., Matsuda index |
Intravenous glucose tolerance test with minimal model assessment |
(b) Exogenous insulin |
Insulin tolerance test |
Open-loop steady-state tests |
Insulin suppression test |
Hyperinsulinaemic-euglycaemic clamp |
All of the commonly used methods focus primarily on the assessment of glucose metabolism. In the context of developing new drugs for diabetes, this approach is, of course, entirely appropriate. However, the complex dynamic interconnections that exist between carbohydrate, lipid, and protein metabolism support consideration of a broader perspective [101]. We propose that such an approach might prove useful in diabetes drug development. For example, in animal models the fibrate class of lipid-modifying drugs improve glucose metabolism via effects on PPAR-α receptors [102]. In 2008 the bile-acid sequestrant colesevelam was licensed by the US Food and Drug Administration (FDA) for the adjunctive treatment of hyperglycaemia in patients with type 2 diabetes. While the glucose-lowering mechanisms of this lipid-modifying agent have yet to be clarified, improved hepatic insulin sensitivity has been reported [103]. Selective cannabinoid receptor agonists [104, 105] and novel PPAR-γ agonists [106] have effects on multiple facets of intermediary metabolism that may contribute to their glucose-lowering actions.
Minimizing Confounding Factors in Studies of Insulin Sensitivity
When designing clinical studies evaluating novel insulin-sensitizing drugs or insulin preparations, confounding factors that may impact insulin sensitivity should be carefully considered (Table 1.7). Subjects should be maintained on a stable diet with constant exercise levels and should be free of acute illness or a history of recent hospitalization. These factors, if not held constant as far as possible, may contribute to day-to-day variability in insulin sensitivity thereby reducing the statistical power of the study. Admission to the clinical facility a day or two ahead of glucose clamp study days is recommended in order to stabilize diet, exercise, and sleep patterns [100, 107]. A practical consideration is the residual effect of prior treatment with drugs affecting glucose metabolism or body weight. In countries with well-developed healthcare systems, it may be difficult to recruit drug-naïve patients with type 2 diabetes since metformin is recommended at an early stage after diagnosis [49]. Monotherapy may be withheld from suitable patients with type 2 diabetes in preparation for early-phase studies of an experimental diabetes drug (see Chap. 9). For thiazolidinediones [108] and glucagon-like peptide (GLP)-1 receptor agonists [109] which have sustained effects on insulin sensitivity and/or body weight, a sufficient period of withdrawal is recommended to ensure a new steady state.
Table 1.7
Factors that may influence insulin sensitivity of relevance to metabolic studies of insulin action
Ethnicity (e.g., East and South Asians may be insulin resistant even in the absence of generalized obesity) |
Body weight should be stable (within 5–10 %) in the weeks preceding a metabolic study. For in-house studies, care must be taken to maintain body weight |
Family history of type 2 diabetes may be associated with greater degrees of insulin resistance |
Personal history of glucose intolerance (e.g., gestational diabetes; an insulin-resistant state carrying a high risk of progression to permanent type 2 diabetes) |
Recent intercurrent illness (moderate inflammatory response and/or tissue damage/repair are associated with reduced insulin sensitivity via release of cytokines) |
History of recent (within 6–8 weeks of study) major surgery (includes minimally invasive procedures requiring a general anaesthetic |
Current or recent history of malignant disease |
Polycystic ovary syndrome (relatively common among women of reproductive age) |
Male hypogonadism |
Impaired function of major organs, i.e. heart, liver, kidney – see Table 1.1 |
Medications; many drugs may affect insulin sensitivity and glucose tolerance |
Diet – calorie consumption, macronutrient composition, and micronutrient intake may each independently influence insulin sensitivity |
Recent acute physical exertion (avoid rigorous exercise for >24 h prior to metabolic studies) |
Alcohol consumption (U-shaped association with insulin resistance; excess alcohol consumption may aggravate hypertriglyceridaemia; avoid alcohol for 24 h prior to study) |
Tobacco (use associated with insulin resistance; smoking activates sympathetic nervous system activity) |
Caffeine (high caffeine consumption may acutely reduce insulin sensitivity; in contrast, habitual coffee consumption is protective against the development of type 2 diabetes) |
Disorders associated with antagonism of insulin action, e.g., hyperthyroidism, hypothyroidism, hyperprolactinaemia |
Rare inherited or acquitted syndromes, e.g., lipoatrophic diabetes, insulin receptor antibody syndromes |
Closed-Loop Assessments
Tests of Basal Insulin Sensitivity
Fasting Insulin Levels
In the fasting state hyperinsulinaemia in the presence of normo- or hyperglycaemia is indicative of insulin resistance. Homeostasis model assessment (HOMA) is a mathematical model that estimates insulin sensitivity as a percentage of a normal population based on fasting serum insulin and blood-glucose concentrations.
HOMA-IR
This test was originally described by David Matthews and colleagues at the University of Oxford [110]. Both the original HOMA approach and the updated version (HOMA2) [111] assume the presence of a feedback loop between the liver and β-cells. HOMA-IR is the reciprocal of %S (100/%S).
G b and I b are, respectively, basal (fasting) glucose (in mmol/L) and insulin (in mU/L) with k = 22.5.

Since physiological insulin secretion from the β-cells is pulsatile, the mean of three samples taken at 5 min intervals to compute HOMA may be more accurate than a single sample. In practice a single measurement is often used and for large sample sizes, this compromise provides comparable data [112]. Since insulin action is not measured directly, HOMA-IR may be more appropriately considered a surrogate of insulin resistance. A similar approach, the quantitative insulin-sensitivity check (QUICKI), is the reciprocal of the logarithm of HOMA-IR with k assigned a value of 1 [113]. In this model, logarithmic transformation accounts for the nonnormal distribution of fasting serum insulin concentrations. HOMA has been compared with a variety of investigative methods for assessing insulin sensitivity, including the glucose clamp [112], albeit with varying conclusions [114]. It has been proposed that HOMA-IR provides information that is primarily relevant to basal hepatic glucose metabolism. Thus, if insulin-stimulated glucose uptake in skeletal muscle is of interest, consideration should be given to using a technique that raises circulating insulin concentrations. The insulin-glucose HOMA model cannot be used to assess β-cell function in patients taking exogenous insulin.
HOMA-IR is technically straightforward, quantitative, and relatively inexpensive. Accuracy is dependent in part on the precision of the insulin assay as well as the type of sample, i.e. serum vs. plasma [115]. The absence of an international standardized insulin assay [116] precludes use of HOMA-IR to define universal cut-off points for insulin resistance. For subjects without diabetes, the correlation between HOMA-IR and fasting insulin concentration is close to unity [117]. HOMA-IR is well suited to large-scale studies where it is perhaps most appropriately positioned [118]. The relatively high intra-individual variability of HOMA-IR renders the index less suitable for determining the impact of an intervention on insulin sensitivity in early-phase studies in patients with type 2 diabetes [119]. The correlations between HOMA and a more sophisticated measure of insulin-mediated glucose uptake were less good in normal weight individuals than in overweight or obese subjects (correlation coefficients 0.36, 0.55, and 0.60, respectively [117]. Similar (inverse) trends were observed for QUICKI [117].
HOMA-IR may be of value as an exploratory endpoint in interventional studies where a change in insulin sensitivity is postulated. For example, a study of the effects of niacin/laropiprant in women with ovary syndrome showed an impairment of glucose regulation despite beneficial effects on blood lipid profiles. The adverse effect on glucose metabolism was accompanied by an increase in HOMA-IR (3.8 vs. 2.2; p = 0.02) indicating reduced insulin sensitivity [120]. In a meta-analysis of nondiabetic patients, angiotensin receptor blockers improved HOMA-IR relative to calcium channel blockers for a similar degree of blood pressure lowering [121]. These effects on insulin sensitivity may be of relevance to the hierarchy of risk of new-onset type 2 diabetes associated with the use of different classes of antihypertensive agents [122].
Closed-Loop Dynamic Tests
An oral glucose or mixed-meal challenge can be used to provide indirect or surrogate information about insulin resistance under more physiological conditions. In both scenarios, the release of endogenous insulin is stimulated by intestinally absorbed glucose in part via the activation of the incretin system [123]. In the situation wherein fasting and/or postprandial hyperglycaemia are present, the degree of hyperinsulinaemia will underestimate the level of insulin resistance. If compensatory endogenous insulin secretion were to be restored, e.g. by use of a classic insulin secretagogue drug, such as a sulphonylurea, then the resulting hyperinsulinaemia would return blood-glucose levels towards normality. In the case of sulphonylureas, the dynamics of insulin secretion are not normalized in an important sense; insulin secretion is not regulated by prevailing glucose concentrations [50]. Sulphonylureas will stimulate insulin secretion even when plasma glucose is normal with a well-recognized risk of hypoglycaemia. The risk varies according to the sulphonylurea [124]. The risk of inappropriate hyperinsulinaemia is largely avoided with glucagon-like peptide (GLP)-1 agonists and dipeptidyl peptidase (DPP)-4 inhibitors [50, 125]. This recently introduced class of incretin mimetics promotes insulin secretion only in the presence of hyperglycaemia [125].
Intravenous Glucose Tolerance Test
This technique, in which the caveat concerning impaired β-cell function also applies, has been widely applied in clinical metabolic research. A bolus of glucose (0.3 g/kg) is administered via an indwelling venous catheter after an overnight fast. Venous blood is sampled frequently from the contralateral arm for insulin and glucose over a period of 3 h [126]. The peak, and subsequent decline, in blood glucose reflects both the insulin response and whole-body insulin sensitivity. Modifications of the frequently sampled intravenous glucose tolerance test (FSIVGTT) include co-administration of tolbutamide to enhance insulin secretion [127] or a bolus of exogenous insulin (0.5 U/kg) [18]. Richard Bergman and colleagues advanced the analysis of data derived from the FSIVGTT and modified FSIVGTT with the introduction of the so-called minimal model [128, 129]. The insulin-sensitivity index, SI, is calculated from two differential equations. SI, which reflects composite insulin action on muscle and adipose tissue, reportedly correlates well with the M-value obtained using the hyperinsulinaemic-euglycaemic glucose clamp technique, at least in relatively insulin-sensitive and glucose-tolerant subjects [130]. In addition, the minimal model permits an assessment of the ability of glucose to promote its own disappearance from the circulation (and inhibit its endogenous appearance) independently of insulin; this value is known as glucose effectiveness or SG [131]. The FSIVGTT avoids potential variations in gastric emptying that may affect the metabolic response to an oral glucose challenge in patients with diabetic autonomic neuropathy [132]. However, this advantage comes at the expense of losing the contribution of the powerful incretin effect on insulin secretion [133].
Concerns have been expressed about aspects of the validity of the minimal model and its limited correlation with more direct methods for assessing insulin action [134–136]. Nonetheless, using the theoretical framework of the minimal model, Michael Schwartz and colleagues at the University of Washington, USA have recently proposed the development of novel diabetes therapies that target a putative brain-centred glucoregulatory system modulating insulin-independent mechanisms (SG) [137].
Oral and Mixed Meal Tolerance Tests
The oral route of glucose delivery is self-evidently more physiological than an intravenous glucose infusion. However, the oral glucose tolerance test (OGTT) cannot be regarded as a physiological stimulus, even though this is sometimes implied in the literature. The OGTT is recognized as having relatively low day-to-day reproducibility [138]. Factors contributing to intra-individual variability include inconstant rates of glucose absorption and splanchnic glucose uptake, variations in gastric emptying including gastroparesis due to autonomic neuropathy in some patients [139], and the modulating effects of gut-derived incretin hormones A longer-term influence on glucose tolerance is the macronutrient composition of the diet, e.g. percentage of calories derived from carbohydrate vs. fat and the proportion of monounsaturated fat [140]. Less well-documented dietary factors include micronutrient status [141] and the influence of the intestinal microbiome [142].
The 75 g OGTT is widely used in clinical practice to confirm diagnostic categories of glucose intolerance and type 2 diabetes [143]. After overnight fast, venous blood samples for glucose and insulin concentrations are taken at baseline and then every 30 min until 120 min following a standard oral glucose load (75 g); in a mixed meal tolerance test a standardized meal or meal substitute, e.g. Ensure®, may be used for clinical research purposes. The diagnosis of impaired glucose intolerance and diabetes are based solely on the baseline and 120 min blood-glucose levels [143]. Note that the classification limits differ according to the sample used, e.g. venous plasma vs. whole blood. Glucose should be dissolved in water so that the maximum glucose concentration in the beverage is 25 g per 100 mL. The drink, which may be made more palatable with a non-calorie flavour additive, should be consumed within 5 min with the subject sitting quietly throughout the test. For children, the glucose load is calculated according to body weight, i.e. 1.75 g per kg of weight to a maximum of 75 g. It has long been recognized that dietary carbohydrate restriction in the days preceding an OGTT may impair glucose tolerance. Accordingly, 100–150 g/day carbohydrates should be consumed as part of the diet for 3 days prior to the scheduled OGTT. In parentheses, the clinical impact of carbohydrate intake on insulin action is illustrated by the benefits of oral carbohydrate loading on surgical outcomes [144].
The OGTT (and meal tolerance test) mimics the glucose and insulin dynamics of physiological conditions more closely than the insulin suppression test, FSIVGTT, or hyperinsulinaemic-euglycaemic glucose clamp. Several insulin-sensitivity indices based on the OGTT are available. These include the Matsuda index [145] and the Stumvoll index [146]. The latter has been validated against the glucose clamp technique in subjects with a range of metabolic states and is widely regarded as a reliable indicator of insulin sensitivity. Others include the OGIS120 index of Mari et al. [147] and an oral glucose minimal model enabling measurement of insulin sensitivity that has been developed and validated against multi-tracer and hyperinsulinaemic-euglycaemic glucose clamp protocols [136, 148]. In general, these indices of insulin sensitivity are more accurate in nondiabetic individuals with normal β-cell function. Moving beyond measures of insulin and glucose, Beysen et al. developed the deuterated-glucose disposal test (2H-GDT). The stable isotope [6,6-2H2]glucose is administered in a 75 g oral glucose load in order to determine whole-body glycolysis. Glycolytic disposal of the deuterated glucose generates 2H2O from which an index of insulin sensitivity is calculated using the insulin exposure resulting from the glucose challenge [149]. The investigators report close correlations with measures of insulin resistance using the hyperinsulinaemic-euglycaemic glucose clamp and the insulin suppression test (see below) across a range of insulin sensitivities [149].
The rise in blood glucose following oral loading is determined in part by the degree of suppression of hepatic glucose production in addition to the absorption and disposal of the oral glucose load [150]. Radiolabelled tracers may be used to ascertain the metabolic fate of an intestinal glucose load and quantify the contribution of endogenous glucose production to the post-challenge blood-glucose concentration. The merits of the various tracer options, which involve use of two or three ingested and infused tracers, have recently been reviewed [151]. As an example of the application of tracer methodology in a proof-of-mechanism study, Polidori et al. used a mixed-meal tolerance test with a dual-tracer (3H-glucose14,C-glucose) method to examine the effects of canagliflozin, a sodium-glucose cotransporter (SGLT)-2 inhibitor which also has activity at the intestinal SGLT-1 receptor. Canagliflozin reduced postprandial plasma glucose and insulin levels in healthy subjects by increasing urinary glucose excretion (via renal SGLT-2 inhibition) and by delaying the rate of appearance of oral glucose (RaO), the latter being attributed to intestinal SGLT-1 inhibition [152]. In this study, plasma insulin level was reduced as a secondary consequence of reduced intestinal glucose absorption [153]. This study provides an illustration of the well-recognized phenomenon whereby an improvement in insulin sensitivity, indicated by lower insulin levels in conjunction with improved post-challenge glucose tolerance, may occur secondary to a reduction in hyperglycaemia achieved by other means. This is an important general point that should be borne in mind since any intervention that lowers blood glucose such as dietary modifications or exercise, and a range of non-insulin-sensitizing drugs, can reduce insulin resistance by relieving the negative metabolic impact of hyperglycaemia i.e. glucotoxity [48]. Determining whether a drug exerts a primary insulin-sensitizing effect requires direct evidence of improved insulin action using appropriate investigative techniques. Of these, the hyperinsulinaemic-euglycaemic glucose clamp is regarded as the reference method (see below).
Insulin Tolerance Test (ITT)
This involves an intravenous injection of a bolus of exogenous insulin (typically 0.1 U/kg) in the fasting state. The response of blood glucose reflects the combined effects of the injected insulin on hepatic and peripheral insulin-sensitive tissues. Due to the dynamic rise and subsequent fall in serum insulin concentrations, the contribution to the decline in blood glucose by reduced hepatic glucose production and insulin-stimulated glucose uptake, respectively, will vary during the test according to relative dose-response characteristics (Fig. 1.3). An insulin-sensitivity index may be calculated from the ratio of the change in blood glucose to the basal glucose concentration. The glucose disposal rate (KITT) may be calculated from the slope of the regression line of the logarithm of blood glucose against time during the first 3–15 min [154]. The ITT has important limitations, notably the risk of inducing hypoglycaemia. Clinical hypoglycaemia is usually unpleasant and is potentially hazardous in certain subjects, e.g. patients with ischaemic heart disease. Furthermore, the release of the counterregulatory hormones, primarily glucagon and catecholamines antagonizes the actions of insulin confounding the test results. A mean coefficient of variation of 30 % for the ITT was reported in a study using 0.05 U/kg insulin healthy volunteers [154]. However, better reproducibility has been reported using the same insulin dose by other investigators [155]. The ITT is not widely used in clinical metabolic research nor is the technique regarded as meeting the requirements for drug development studies.
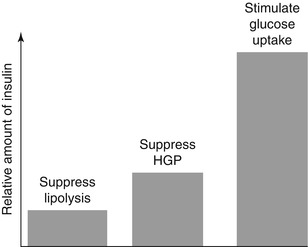
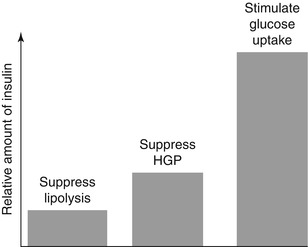
Fig. 1.3
Approximate relative amounts of insulin required for maximal effects on major metabolic processes in vivo. HGP hepatic glucose production
Open-Loop Methods
Insulin Suppression Test (IST)
This method, introduced by Gerald Reaven and colleagues at Stanford University, USA in 1970, was the first to offer a direct measurement of insulin action in human subjects [136, 156]. In its original inception, an intravenous infusion of adrenaline (epinephrine) was used to suppress endogenous insulin secretion. The non-selective β-adrenergic blocker propranolol was co-infused to counter the metabolic and haemodynamic effects of the adrenaline. However, complete blockade of the adrenergic receptors could not be guaranteed and the hazard of cardiac arrhythmias led to a modification of the approach by which to interrupt the glucose-β-cell feedback loop [157]. After an overnight fast, an intravenous infusion of somatostatin or the somatostatin analogue octreotide [158] is used to suppress the endogenous secretion of insulin and glucagon from the pancreatic β- and α-cells, respectively [158]. Simultaneous infusions of insulin (25 mU/m2/min) and glucose (240 mg/m2/min) are delivered for 3 h. Blood samples for the determination of glucose and insulin are drawn from the contralateral arm every 30 min for 2.5 h and then at 10 min intervals to 180 min. Under these controlled conditions, the steady-state plasma glucose (SSPG) concentration between 150 and 180 min reflects the net effect of the achieved hyperinsulinaemia on insulin-sensitive tissues. Since SSPG is inversely related to insulin sensitivity, it is predicted that the SSPG concentration will be higher in more insulin-resistant subjects. Assumptions inherent in the interpretation of the IST include the complete suppression of endogenous insulin, glucagon and growth hormone secretion (which is also suppressed by somatostatin), reproducible direct effects of somatostatin on splanchnic blood flow and peripheral glucose metabolism, and attainment of steady-state hyperinsulinaemia. While the IST has been used extensively in clinical metabolic research, it has not been widely applied in diabetes drug development.
Insulin-Sensitivity Clamp
This technique, usually known as hyperinsulinaemic-euglycaemic glucose clamp, is widely regarded as the ‘gold standard’ among methods for quantifying insulin action. However, while this accolade attests to advantages that the technique has over some of the alternatives, it should not be taken to imply that the glucose clamp technique offers unique insights into insulin action that more fundamentally reflect human physiology. The hyperinsulinaemic-euglycaemic glucose clamp technique is a pharmacological perturbation of homeostatic metabolic mechanisms that provides quantifiable and reproducible data with which to test hypotheses concerning the role of insulin resistance in human disease.
The hyperinsulinaemic-euglycaemic glucose clamp establishes a temporary state of sustained hyperinsulinaemia. This permits quantification of a key aspect of glucose metabolism, i.e. insulin-mediated glucose disposal. One of the advantages of the glucose clamp technique is that it be can be readily adapted to provide a broader perspective of human metabolism, including assessments of hepatic glucose production and lipolysis. When selecting target insulin levels it should be noted that the inhibitory effect of insulin on hepatic glucose production requires lower plasma insulin concentrations than those required to maximally stimulate glucose uptake in skeletal muscle (Fig. 1.3) [129, 159, 160]. In a one-step hyperinsulinaemic-euglycaemic glucose clamp study performed in healthy volunteers, Rizza et al. observed half-maximal suppression of hepatic glucose production (determined using [3-3H]glucose as a tracer) at a mean (±standard deviation) plasma insulin concentration of 174 ± 12 pmol/L (29 ± 2 mU/L). In contrast, the insulin concentration required for half-maximal stimulation of glucose utilization was nearly twice as high at 330 ± 36 pmol/L (55 ± 7 mU/L) [161]. Maximal glucose utilization occurred at pharmacological insulin concentrations of 1,320–4,200 pmol/L (220–700 mU/L). Other groups have reported similar findings. As discussed above, impaired insulin-mediated suppression of hepatic glucose production, i.e. hepatic insulin resistance, is the main driver of pathological states of fasting hyperglycaemia. Accordingly, assessment of liver glucose output is of interest in the context of the development of new diabetes drugs.
Following an overnight fast, plasma glucose concentrations are maintained entirely from endogenous sources. The liver and the kidney are the only organs in the human body with sufficient gluconeogenic enzyme activity and glucose-6-phosphatase to release glucose into the circulation via gluconeogenesis. Glucose production by the liver accounts for approximately 80 % with renal glucose production accounting for the remainder [162, 163]. When the insulin sensitivity of glucose metabolism is impaired, other defects are detectable using appropriate techniques [164, 165]. Lipolysis is very sensitive to inhibition by insulin, with only small increments above fasting levels being sufficient to restrain hydrolysis of trigly-cerides (see Fig. 1.3) [160, 164, 165]. Circulating levels of nonesterified (‘free’) fatty acids are often elevated in subjects with obesity, impaired glucose tolerance, and in patients with type 2 diabetes and fail to suppress normally in response to insulin [164]. These defects are indicative of insulin resistance within adipocytes.
Two-Step Hyperinsulinaemic-Euglycaemic Glucose Clamp
This variant can be used to assess direct suppression of fatty acids by insulin and other hormones, e.g. insulin-like growth factor)-1, at low and high insulin concentrations [166]. The multistep hyperinsulinaemic glucose clamp technique may also be combined with isotopic determination of glycerol turnover to provide an alternative measure of lipolysis [167]. The glucose clamp technique is suitable for pairing with various complementary methods that permit insulin action to be studied under controlled conditions at whole-body, regional, or tissue level (Table 1.8). For example, the hyperinsulinaemic-euglycaemic glucose clamp may be performed in conjunction with indirect calorimetry to quantify substrate oxidation. While combining these two methods is conceptually intriguing and if done properly might can generate useful data, it has to be pointed out that managing this sophisticated experimental setup is demanding and requires a high level of expertise and experience. Using this approach, a study of pioglitazone in insulin-resistant women with polycystic ovary syndrome demonstrated that increases in circulating levels of the insulin-sensitizing cytokine adiponectin correlated closely with improvements in glucose and lipid oxidation as well as inversely with changes in fasting fatty acid concentrations [168]. The investigators considered that their observations provided support for the hypothesis that improvements in multiple aspects of insulin sensitivity with pioglitazone were at least partly explained by an increase in adiponectin levels [168]. The flexibility of the glucose clamp readily permits the design of studies in which multiple target glucose levels can be achieved. This approach has been used, for example, to test new glucose sensors [169].
Table 1.8
Examples of complementary investigative methods that may be combined with the hyperinsulinaemic-euglycaemic clamp technique
Method | Measure of interest |
---|---|
Isotopic glucose tracer | Glucose turnover (RaG, RdG) |
Indirect calorimetry | Substrate oxidation |
Magnetic resonance spectroscopy | Intramyocellular lipid; hepatic lipid content |
Positron emission tomography | Regional brain/heart glucose metabolism |
Venous occlusion plethysmography | Endothelial function |
Isotopic glycerol tracer | Lipolysis |
Tissue biopsy (muscle, fat) | Insulin-responsive enzyme expression |
Microdialysis | Adipose tissue substrate metabolism |
Hypoglycaemic Glucose Clamp
This technique, which does not quantify insulin action, is widely used to assess counterregulatory hormone responses under standardized conditions of experimental hypoglycaemia [170]. This design variation of the glucose clamp will usually be based on administering individualized intravenous insulin infusions that will be titrated to the point of achieving a predefined hypoglycaemic blood glucose target, or if designed as a stepwise hypoglycaemic glucose clamp, to achieve and maintain for a certain period of time, e.g. 30 min, several euglycaemic and hypoglycaemic target levels in sequence, e.g. 7, 5, 4, and 3 mmol/L. At each target level blood samples to assess variables of interest, e.g. counterregulatory hormones, incretins, can be collected. At the end of the lowest clamp target level, the intravenous insulin infusion is terminated and the time to spontaneous recovery to euglycaemic blood-glucose levels can be captured as an additional outcome variable.
Islet Cell Clamp
In this variant of the clamp approach, somatostatin or octreotide is infused to suppress endogenous insulin secretion [171]. Target steady-state circulating insulin and glucagon levels may be achieved by appropriate intravenous infusions of theses hormones. Growth hormone, secretion of which from the pituitary is also suppressed by somatostatin, is replaced at basal levels. The islet cell (also known as the pancreatic) clamp can be combined with the hyperglycaemic glucose clamp technique thereby being of particular value for assessing urinary glucose excretion [172].
In another variant, Tonelli et al used the islet cell clamp to investigate the effects of pioglitazone on glucose kinetics in patients with type 2 diabetes during low and high insulin infusion rates at euglycaemia [173]. While endogenous glucose production was suppressed by pioglitazone at both levels of hyperinsulinaemia glucose disposal was increased only during the high insulin infusion rate.
Hyperglycaemic Glucose Clamp
This technique is primarily a method used to evaluate endogenous insulin secretion. However, it can be also be used to measure insulin sensitivity [136] and to assess urinary glucose excretion [95, 172]. A variable quantity of hyperosmolar glucose is infused intravenously to establish predefined levels or a single level of hyperglycaemia, for periods of time during which blood or urine samples are collected in order to determine endogenous insulin secretion or urinary glucose excretion, respectively. The amounts of intravenous glucose needed to establish predefined levels of hyperglycaemia can vary widely between individuals according to insulin sensitivity and the ability of hyperglycaemia per se to enhance glucose uptake [174]. The method, when applied in healthy subjects, also has very practical limitations in that above certain hyperglycaemic target levels, e.g. 10–11 mmol/L or higher, the volume of intravenous glucose needed to establish and then maintain the target level can be excessive due to the ever-increasing concentrations of insulin stimulated by the hyperglycaemia.
The versalility of the glucose clamp technique is illustrated by a recent study in which a pancreatic and stepped hyperglycaemic glucose clamp (plasma glucose range 5.5–30.5 mmol/L) were combined to characterise major components of renal glucose reabsorption in response to dapagliflozin in healthy subjects and patients with type 2 diabetes [172]. Patients with type 2 diabetes (n = 12) and matched healthy subjects (n = 12) were studied at baseline and after 7 days of treatment with dapagliflozin. A pharmacodynamic model was developed to describe the major components of renal glucose reabsorption for both groups and then used to estimate these parameters from individual glucose titration curves. At baseline, the patients with diabetes had elevated maximum renal glucose reabsorptive capacity (TmG), splay (the rounding of the glucose reabsorption curve), and renal threshold compared with controls. Dapagliflozin reduced the TmG and splay in both groups. The most significant effect of dapagliflozin was a reduction of the renal threshold for glucose excretion in the patients with type 2 diabetes and the controls. It was concluded that dapagliflozin increases urinary glucose excretion in patients with type 2 diabetes by reducing the TmG and the threshold at which glucose is excreted in the urine [172].
Experimental Procedure for the Hyperinsulinaemic-Euglycaemic Glucose Clamp
This glucose clamp method, whether comprising a single step or two steps of steady-state hyperinsulinaemia, is conducted in the morning after an overnight fast. An arm vein is cannulated for the infusion of insulin and glucose. In the contralateral forearm, another cannula is placed for sampling, the hand being enclosed in a thermostat-controlled warming unit at approximately 55 °C to open arteriovenous channels and so provide ‘arterialized’ blood that approximates the arterial supply; this avoids the reduction in plasma glucose due to extraction during the passage of blood through insulin-sensitive tissues and so avoids potential overestimation of insulin action [134]. However, hand warming may induce peripheral vasodilatation resulting in a rise in heart rate and changes in blood pressure [175]. Soluble insulin or a rapid-acting insulin analogue is infused using a precision pump at a rate calculated to elevate serum insulin concentration from basal levels to a predefined target within the euglycaemic range, i.e. to approximately 4–5 mmol/L. A typical insulin infusion dose is 1.0 mU/kg/min (6 pmol/kg/min) or 40 mU/min/m2 body surface area (0.24 nmol/min/m2). Insulin doses based on surface area are preferred for obese subjects (body mass index >30 kg/m2) in order to avoid over-insulinization [134]. Infusing insulin at a rate of 1 mU/kg/min generally provides plasma insulin levels in the high physiological range, albeit with wide variations reported in the literature (see below). Adsorption of insulin to the plastic surfaces of the infusion tubing can be avoided by adding 2 mL of the subject’s blood to the insulin/saline solution used for infusion [176].
The response of the key outcome measure, i.e. glucose disposal rate (also known as the M-value), is approximately linear over the physiological range of plasma insulin concentrations (see Fig. 1.2). It has been suggested that very high doses of insulin, i.e. producing pharmacological serum insulin concentrations of 200 mU/L (1,200 pmol/L) or more, carry the risk of saturating the physiological clearance of insulin leading to unpredictable hyperinsulinaemia. However, dose-response effects have been described using doses as high as 5.0 U/kg/min in insulin-resistant subjects [177]. Some centres advocate the use a primed-continuous infusion of insulin to achieve the desired level of hyperinsulinaemia more rapidly, accepting a temporary overshoot above target for this advantage.
Manual vs. Automated Glucose Clamp
During the glucose clamps plasma, glucose is monitored online at intervals ranging from 1 to 10 min. For the purposes of a hyperinsulinaemic glucose clamp, a precise and accurate glucose oxidase or hexokinase reference method which meets requisite quality control standards is required [178]. In response to hyperinsulinaemia, glucose starts to decline as hepatic glucose production is inhibited and, at higher insulin concentrations, glucose uptake is stimulated. Glucose (20 % v/v) is therefore infused intravenously to maintain the arterialized blood glucose constant at the target level. In an isoglycaemic glucose clamp, a pre-existing level of plasma glucose for the individual is selected as the target. In patients with diabetes, blood-glucose levels may be normalized overnight before the studies by a variable rate low-dose intravenous insulin infusion [179]. This approach is valuable for standardizing the baseline metabolic state prior to the clamp in studies in which a therapeutic intervention is tested. While published algorithms for calculating glucose infusion rates during clamps are available [95], many investigators prefer to rely on their own judgment – the so-called manual clamp.
An alternative to the manual clamp is provided by the automated clamp using the Biostator® glucose-controlled insulin infusion system (Life Science Instruments, Elkhart, IN, USA; Fig. 1.4). The Biostator was originally developed as an extracorporeal artificial pancreas [180, 181] but failed to fulfil its clinical potential due to degradation of insulin by the mechanical pump [182]. The device was repositioned for clinical metabolic research as an automated method for performing glucose clamps [183, 184]. In brief, the Biostator senses plasma glucose every minute and adjusts an intravenous infusion of glucose according to an algorithm originally described by Clemens et al. [185]. A double-lumen venous catheter is used for continuous glucose measurement: one tube administers saline-heparin solution at a constant rate and is then mixed and returned with the blood that is withdrawn at an equal rate via the other (inner) lumen. The Biostator algorithm takes the current measurement of blood glucose and the variations in glucose levels during preceding minutes into consideration. In addition to its application in the setting of the hyperinsulinaemic-euglycaemic glucose clamp, the Biostator has been used extensively to determine time-action profiles of glucose-lowering drugs (see below) [186]. Blood glucose is measured continuously by a glucose oxidase sensor. This permits rapid alterations of the glucose infusion rates. However, periodic recalibration of the glucose measurement is required using an external reference method due to inherent drift of the glucose sensor, which is in constant contact with sampled blood. Advantages of the Biostator compared to a manual euglycaemic glucose clamp include relatively small blood volume requirements for measurement of glucose and minute-by-minute adjustment of glucose infusion rates. Perhaps the most compelling advantage that the Biostator offers is the removal of risk of any unconscious bias on the part of the clamp operator [186]. The cost of automated clamps compared to manual clamps is higher only if consumable materials are considered, mainly arising from the expense of glucose sensor membranes and other materials, e.g. the double-lumen sampling catheter. Balanced against these costs are the better quality of the clamp data (=smaller swings of glycaemia around the target values, expressed as the coefficient of variation (CV) of glycaemia), which is unbiased by a human operator, and the fact that a single human operator can manage more than one automated glucose clamp in parallel. Biostator devices have to be carefully maintained and require expertise in application. Second-generation proprietary automated glucose clamp technology is now available that allows an even tighter management of the target glucose concentration to CVs of <5 % and provides results immediately ready for data processing.
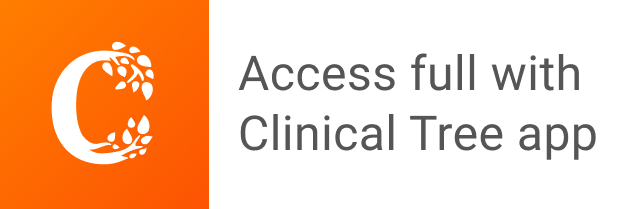